1 Principles of Cell Function
|
The human body is composed of billions of cells, each with a distinct function. Despite this diversity in cell function, all cells share certain common elements and functions. This chapter provides an overview of these common elements and focuses on the important function of transport of molecules and water into and out of the cell across its plasma membrane.
|
OVERVIEW OF EUKARYOTIC CELLS
|
Eukaryotic cells are distinguished by the presence of a membrane-delimited nucleus. With the exception of mature human red blood cells, all cells within the body contain a nucleus. The cell is therefore effectively divided into two compartments: the nucleus and the cytoplasm. The cytoplasm is an aqueous solution containing numerous organic molecules, ions, cytoskeletal elements, and a number of organelles. A brief description of the components of a typical eukaryotic cell follows (Fig. 1-1). Readers who desire a more in-depth presentation of this material are encouraged to consult one of the many cellular and molecular biology textbooks currently available.
|
The nucleus contains the genome of the cell, which in somatic cells is present on 46 chromosomes, 22 pairs of autosomes and one pair of sex chromosomes. Both sperm and eggs contain 23 chromosomes, a copy of each autosome and either a male (X) or a female (Y) sex chromosome. The chromosome is a highly ordered structure containing genes (DNA) and associated proteins (i.e., histones). The nucleus also contains the enzymatic machinery for repair of damaged DNA and for its replication, as well as the enzymes needed to transcribe DNA and yield messenger RNA (mRNA).
|
The plasma membrane surrounds the cell and separates the contents of the cell from the surrounding extracellular fluid. It serves a number of important functions and is described in greater detail later in the chapter.
|
It is currently thought that mitochondria evolved from an aerobic prokaryote that lived within primitive eukaryotic cells. Mitochondria synthesize ATP and thus provide the energy needed to power many vital cell functions. They contain their own DNA, which codes for a number of the enzymes needed for oxidative phosphorylation (other mitochondrial enzymes are synthesized in the cytoplasm and imported into the mitochondria), as well as the RNA needed for the transcription and translation of mitochondrial DNA. Mitochondria are composed of two membranes separated by an intermembrane space. The outer mitochondrial membrane lets molecules up to 5 kDa in size cross. Thus, the composition of the intermembrane space is similar to that of cytoplasm with respect to small molecules and ions. The inner membrane is folded into numerous cristae and is the site where ATP is generated through the process of oxidative phosphorylation. The interior of mitochondria (i.e., matrix) contains the enzymes involved in the citric acid cycle and those involved in oxidation of fatty acids. In addition to producing ATP, mitochondria can serve as a site for sequestration of Ca++.
|
Rough Endoplasmic Reticulum
|
The rough endoplasmic reticulum (rER) is an extensive membrane network throughout the cytoplasm and is especially well developed in cells that produce and secrete proteins (e.g., pancreatic acinar cell, plasma cell). Attached to the membrane are ribosomes, which when viewed with an electron microscope, impart the "rough" appearance characteristic of this organelle. The rER is the site of translation of mRNA and posttranslational modification of proteins that are destined to be secreted from the cell or are targeted to the plasma membrane or other membranous organelles (e.g., Golgi apparatus, lysosomes).
|
Proteins synthesized in the rER are transferred to the Golgi apparatus via coated vesicles. On electron micrographs the Golgi apparatus appears as a stack of flattened membrane sacs. Vesicles from the rER fuse with sacs that are in close proximity to the rER (i.e., the cis-Golgi network). The proteins then traverse through the Golgi membrane sacs, also via coated vesicles, and in this process they may undergo additional posttranslational modification (e.g., glycosylation). The Golgi apparatus also sorts the proteins and packages them for delivery to other parts of the cell (e.g., plasma membrane, lysosome, secretory granule). The sorting and packaging of proteins occur in the trans-Golgi network.
|
Smooth Endoplasmic Reticulum
|
page 3 |  | page 4 |
Figure 1-1 Schematic drawing of a eukaryotic cell. The top portion of the cell has been removed to illustrate the nucleus and various intracellular organelles. See text for details. |
The smooth endoplasmic reticulum (sER) is devoid of ribosomes and therefore appears "smooth" on electron micrographs. It is a site where many substances are modified and detoxified (e.g., pesticides). Hydrophobic molecules can be converted to water-soluble
molecules in the sER, thus facilitating their excretion from the body by the liver and kidneys. The sER is also the site for the synthesis of fats and lipids. For example, the cells of the adrenal gland that secrete the steroid hormone cortisol have an extensive sER. Similarly, the cells within the ovaries and testes that secrete estrogens and testosterone have a well-developed sER. In skeletal and cardiac muscle, the sER, which is called the sarcoplasmic reticulum in these cells, serves to sequester Ca++. Thus, it plays an important role in controlling contraction.
|
Lysosomes are part of the endocytic system of the cell (see later) and serve a degradative function. They are membrane-bound organelles with an acidic interior (pH ≈4.5), and they contain a number of digestive enzymes (e.g., proteases, nucleases, lipases, glycosidases). Lysosomes degrade material that is brought into the cell via the processes of endocytosis and phagocytosis. They also degrade intracellular organelles, a process called autophagy, and some intracellular proteins. Much of what is degraded is then recycled by the cell. The process of degradation is not random and in a number of instances is targeted. For example, chaperone proteins (e.g., heat shock protein 73) can direct intracellular proteins to the lysosome. In addition, plasma membrane proteins can be targeted for endocytosis and eventual degradation by lysosomes through attachment of specific groups (e.g., ubiquitin) to the protein. These groups act as signals for degradation of the protein.
|
Like lysosomes, proteasomes serve a degradative function. However, proteasomes are not membrane bound. They serve to degrade primarily intracellular proteins that have been targeted (e.g., ubiquitinated) for degradation. They may also degrade some membrane-associated proteins.
|
Ribosomes are located throughout the cytoplasm and are not associated with the endoplasmic reticulum. They translate mRNA for cytosolic proteins, as well as proteins that will neither be secreted from the cell nor incorporated into membrane structures (e.g., mitochondrial enzymes).
|
Peroxisomes (also called microbodies) are membrane-bound organelles that contain various oxidative enzymes (e.g., catalase). These oxidative enzymes can detoxify a number of compounds and oxidize fatty acids. In the liver, peroxisomes metabolize ethanol to acetaldehyde.
|
page 4 |  | page 5 |
The cytoskeleton of the cell consists of actin filaments (also called microfilaments), intermediate filaments, and microtubules. Actin filaments in muscle cells are critical components of the contractile apparatus. In other cells they are involved in locomotion (e.g., macrophages). Actin also makes up the core of microvilli and links the interior of the cell to adjacent cells
through some cell junctions (e.g., zonula adherens and zonula occludens). There are several different classes of intermediate filaments, and they can vary by cell type. For example, keratin filaments are found in epithelial cells, whereas neurofilaments are found in neurons. Intermediate filaments are primarily structural in function and can link the interior of the cell to adjacent cells and the surrounding extracellular matrix through desmosomes and hemidesmosomes, respectively. Microtubules serve multiple functions within the cell, including intracellular transport of vesicles, chromosome movement during mitosis and meiosis, and movement of cilia and flagella (e.g., tail of spermatozoa). They are formed from α- and β-tubulin dimers and change length by either adding or removing tubulin dimers. In general, a microtubule-organizing center exists near the cell's nucleus, and microtubules grow out from this center toward the periphery of the cell. As noted, microtubules can move intracellular vesicles within the cell (e.g., transport of neurotransmitter-containing vesicles from the cell body of the neuron down the axon); such movement is driven by motor proteins. One motor protein, kinesin, drives transport from the center of the cell toward the periphery, whereas another motor protein, dynein, drives movement in the opposite direction. Dynein is the motor protein that drives the movement of both cilia and flagella.
|
Microtubules are the target of a number of antitumor drugs (e.g., vincristine and taxol) because disruption of these structures impairs cell division in the highly mitotic tumor cells. Vincristine prevents polymerization of the tubulin dimers and thus prevents the formation of microtubules. As a result, the mitotic spindle cannot form, and the cell cannot divide. Taxol stabilizes the microtubules and thus arrests cells in mitosis. |
Kartagener's syndrome is an autosomal recessive disorder in which dynein is missing in cilia and, in males, the flagella of sperm. Accordingly, males with this syndrome are infertile. Because the cilia of the epithelial cells that line the respiratory track work to remove inhaled pathogens, a process termed mucociliary transport (see Chapter 20), both men and women with this syndrome are susceptible to repeated lung infections. |
 |
The cells within the body are surrounded by a plasma membrane that separates the intracellular contents from the extracellular environment. Because of the properties of this membrane, in particular, the presence of specific membrane proteins, the plasma membrane is involved in a number of important cellular functions, including
- Selective transport of molecules into and out of the cell, a function carried out by membrane transport proteins
- Cell recognition via cell surface antigens
- Cell communication through neurotransmitter and hormone receptors and signal transduction pathways
- Tissue organization, such as temporary and permanent cell junctions, as well as interaction with the extracellular matrix, through a variety of cell adhesion molecules
- Enzymatic activity
- Determination of cell shape by linking the cytoskeleton to the plasma membrane
|
Membranes also surround the various organelles within the cell. The organelle membranes not only subdivide the cell into compartments but are also the site of many important intracellular processes (e.g., electron transport by the inner mitochondrial membrane).
|
In this chapter the structure and function of the plasma membrane of eukaryotic cells is considered. More specifically, the chapter focuses on transport of molecules and water across the plasma membrane. Only the principles of membrane transport are presented here. Additional details as related to specific cells are presented in the various sections and chapters of the book.
|
Structure and Composition
|
The plasma membrane of eukaryotic cells consists of a 5-nm-thick lipid bilayer with associated proteins (Fig. 1-2). Some of the membrane-associated proteins are integrated into the lipid bilayer, whereas others are more loosely attached to the inner and outer surfaces of the membrane, often by binding to the integral membrane proteins. Because the lipids and proteins can diffuse within the plane of the membrane and the appearance of the membrane varies regionally as a result of the presence of different membrane proteins, this depiction of the structure of the plasma membrane is often termed the fluid mosaic model.
|
The major lipids of the plasma membrane are phospholipids or phosphoglycerides. Phospholipids are amphipathic molecules that contain a charged (or polar) hydrophilic head and two (nonpolar) hydrophobic fatty acyl chains (Fig. 1-3). The amphipathic nature of the phospholipid molecule is critical for formation of the bilayer, with the hydrophobic fatty acyl chains forming the core of the bilayer and the polar head groups exposed on the surface.
|
page 5 |  | page 6 |
Figure 1-2 Schematic diagram of the cell plasma membrane. Not shown are lipid rafts. See text for details. (Modified from Figure 12-3 in Cooper GM: The Cell-A Molecular Approach, 2nd ed. Washington DC, Sinauer, 2000.) |
Figure 1-3 Models of the major classes of plasma membrane lipids depicting the hydrophilic and hydrophobic regions of the molecules. The molecules are arranged as they exist in one leaflet of the bilayer. The opposing leaflet is not shown. One of the fatty acyl chains in the phospholipid molecule is unsaturated. The presence of this double bond produces a "kink" in the fatty acyl chain that prevents tight packing of membrane lipids and increases membrane fluidity. (Modified from Hansen JT, Koeppen BM: Netter's Atlas of Human Physiology. Teterboro, NJ, Icon Learning Systems, 2002.) |
Table 1-1.
Plasma Membrane Lipids |
Phospholipid | Leaflet Location |
Phosphatidylcholine | Outer |
Sphingomyelin | Outer |
Phosphatidylethanolamine | Inner |
Phosphatidylserine | Inner |
Phosphatidylinositol* | Inner |
*Involved in signal transduction.
|
The majority of membrane phospholipids have a glycerol backbone to which are attached the fatty acyl chains, as well as an alcohol linked to glycerol via a phosphate group. The common alcohols are choline, ethanolamine, serine, inositol, and glycerol. Another important phospholipid, sphingomyelin, has the amino alcohol sphingosine as its backbone instead of glycerol. Table 1-1 lists these common phospholipids. The fatty acyl chains are usually 14 to 20 carbons in length
and may be saturated or unsaturated (i.e., contain one or more double bonds).
|
The phospholipid composition of the membrane varies among different cell types and even between the bilayer leaflets. As summarized in Table 1-1, phosphatidylcholine and sphingomyelin are found predominantly in the outer leaflet of the membrane, whereas phosphatidylethanolamine, phosphatidylserine, and phosphatidylinositol are found in the inner leaflet. As described in detail in Chapter 3, phosphatidylinositol plays an important role in signal transduction, and its location in the inner leaflet of the membrane facilitates this signaling role.
|
page 6 |  | page 7 |
The sterol molecule cholesterol is also a critical component of the bilayer (Fig. 1-3). It is found in both leaflets and serves to stabilize the membrane at normal body temperature (37°C). Cholesterol can represent as much as 50% of the lipids found in the membrane. Another minor lipid component of the plasma membrane is glycolipid. These lipids, as their name indicates, contain two fatty acyl chains linked to polar head groups that consist of carbohydrates (Fig. 1-3).
As discussed later, one glycolipid, glycosylphosphatidylinositol (GPI), plays an important role in anchoring proteins to the outer leaflet of the membrane. Both cholesterol and glycolipids, like the phospholipids, are amphipathic and orient with their polar groups on the outer surface of the leaflet in which they are located. Their hydrophobic portions are thus located within the interior of the bilayer.
|
The lipid bilayer is not a static structure. Lipids can freely diffuse within the plane of the membrane. The fluidity of the membrane is determined by temperature and by its lipid composition. As temperature increases, the membrane becomes more fluid. The presence of unsaturated fatty acyl chains in phospholipids and glycolipids also increases membrane fluidity. If a fatty acyl chain is unsaturated, the presence of a double bond introduces a "kink" in the molecule (Fig. 1-3). This kink prevents the molecule from closely associating with surrounding lipids, and as a result membrane fluidity is increased. Some membranes contain lipids (e.g., sphingomyelin and cholesterol) that aggregate into what are called lipid rafts. These lipid rafts often have specific proteins associated with them and diffuse in the plane of the membrane as a discrete unit. Lipid rafts appear to serve a number of functions. One important function of these rafts is to segregate signaling mechanisms and molecules.
|
As much as 50% of the membrane is composed of protein. These membrane proteins are classified as either integral, lipid anchored, or peripheral (Fig. 1-2).
|
Integral membrane proteins are embedded in the lipid bilayer, where hydrophobic amino acid residues are associated with the hydrophobic fatty acyl chains of the membrane lipids. Many integral membrane proteins span the bilayer and are termed transmembrane proteins. Transmembrane proteins have both hydrophobic and hydrophilic regions. The hydrophobic region, often in the form of an α helix with the hydrophobic amino acids facing out, spans the membrane. Hydrophilic amino acid residues are then exposed to the aqueous environment on either side of the membrane. Transmembrane proteins may pass through the membrane multiple times.
|
There is a superfamily of membrane proteins that serve as receptors for many hormones, neurotransmitters, and numerous drugs. These receptors are coupled to heterotrimeric G proteins and are termed G protein-coupled receptors (see Chapter 3). These proteins span the membrane with seven α-helical domains. The extracellular portion of the protein contains the ligand binding site, whereas the cytoplasmic portion binds to the G protein. This superfamily of membrane proteins makes up the third largest family of genes in humans. Nearly half of all nonantibiotic prescription drugs are targeted toward G protein-coupled receptors. |
 |
Proteins can also be attached to the membrane via lipid anchors. The protein is covalently attached to a lipid molecule, which is then embedded in one leaflet of the bilayer. The glycolipid GPI anchors proteins to the outer leaflet of the membrane. Proteins can be attached to the inner leaflet via their amino-terminus by fatty acids (e.g., myristate or palmitate) or via their carboxyl-terminus by prenyl anchors (e.g., farnesyl or geranylgeranyl).
|
Peripheral proteins may associate with the polar head groups of the membrane lipids but more commonly bind to integral or lipid-anchored proteins. Peripheral proteins are easily removed from the membrane, whereas integral and lipid-anchored proteins require the use of detergents to isolate them from the membrane.
|
MECHANISMS OF MEMBRANE TRANSPORT
|
Intracellular and extracellular fluid is composed primarily of H2O in which solutes (e.g., ions, glucose, amino acids) are dissolved. The normal function of cells requires continuous movement of water and solutes into and out of the cell. The plasma membrane, with its hydrophobic core, is an effective barrier to the movement of virtually all of these biologically important solutes. It also restricts movement of water across the membrane. With the exception of gases (e.g., O2 and CO2) and ethanol, which can diffuse across the lipid bilayer, movement of water and other solutes across the plasma membrane occurs via specific membrane transport proteins.
|
Membrane Transport Proteins
|
Table 1-2 lists the major classes of membrane transport proteins, their mode of transport, and the rate at which they transport molecules or ions across the membrane.
|
Table 1-2.
Major Classes of Plasma Membrane Transporters |
Class | Transport Mode | Transport Rate |
Water channel | Gated* | Up to 109 molecules/second |
Ion channel | Gated | 106-108 molecules/sec |
Solute carrier | Cycle | 102-104 molecules/sec |
ATP dependent | Cycle | 102-104 molecules/sec |
*Water channels (i.e., aquaporins) may be continuously open and thus function similar to a pore, which is not gated (e.g., the porins found in the outer membrane of mitochondria). However, the permeability of a water channel can be modified and is therefore listed as gated.
|
page 7 |  | page 8 |
Water channels, or aquaporins (AQPs), are the main route for water movement into and out of the cell. They are widely distributed throughout the body, although different isoforms are found in different cell types. To date, 11 AQPs have been identified. The amount of H2O that can enter or leave the cell via AQPs can be regulated by altering the number of AQPs in
the membrane or by changing their permeability (i.e., gating). Changes in pH have been identified as one factor that can modulate the permeability of AQPs.
|
AQPs are divided into two subgroups. One group is permeable only to water. The second group is permeable not only to water but also to low-molecular-weight substances. Because glycerol can cross the membrane via this later group of AQPs, they are termed aquaglyceroporins. AQPs exist in the plasma membrane as a homotetramer, with each monomer functioning as a water channel. |
 |
Ion channels are found in all cells and are especially important for the function of excitable cells (e.g., neurons and muscle cells). Ion channels are classified by their selectivity (i.e., the ions that pass through the channel). At one extreme, they can be highly selective by allowing only a specific ion through. At the other extreme, they may be nonselective and allow all or a group of cations or anions through. Channels are also characterized by their conductance, which is typically expressed in picosiemens (pS). The range in conductance
is considerable, with some channels having a conductance of only 1 to 2 pS and others having a conductance of greater than 100 pS. For some channels, conductance varies depending on which direction the ion is moving. For example, if the channel has greater conductance when ions are moving into the cell versus out of the cell, the channel is said to be an inward rectifier. Finally, ion channels can be classified by their mechanism of gating. As illustrated in Figure 1-4, ion channels fluctuate between an open state or a closed state, a process called gating. Factors that can control gating include membrane voltage, extracellular agonists or antagonists (e.g., acetylcholine is an extracellular agonist that controls the gating of a cation-selective channel in the motor end plate of skeletal muscle cells-see Chapter 12), intracellular messengers (e.g., Ca++, ATP, cGMP), and mechanical stretch of the plasma membrane. Transmembrane ion flux can be regulated by changing the number of channels in the membrane or by gating of the channels.
|
Figure 1-4 Recording of current flow through a single K+-selective ion channel. The channel spontaneously fluctuates between an open and closed state. The amplitude of the current is approximately 2 pA (2 × 10-12 amps), or 12.5 million ions cross the membrane per second. |
page 8 |  | page 9 |
Solute carriers represent a large family of membrane transporters, with more than 40 different types (>300 specific transporters) already identified. These carriers are divided into three major functional groups. One group, uniporters, transports a single molecule across the membrane. The transporter that brings glucose into the cell (GLUT2) is an important member
of this group. The second group, symporters, couples the movement of two or more molecules/ions across the membrane. As the name implies, the molecules are transported in the same direction. Co-transport is another term used to describe this group of solute carriers. The 1Na+,1K+,2Cl- symporter found in the kidney (NKCC2), which is critically important for diluting and concentrating urine (see Chapter 33), is an example of a member of this group. The third group, antiporters, also couples the movement of two or more molecules/ions across the membrane. However, in this case the molecules/ions are transported in opposite directions. The terms exchangers and counter transporters are also used to describe this group of solute carriers. The Na+-H+ antiporter is an example of this group of solute carriers. One isoform (NHE-1) of this antiporter is found in all cells and plays an important role in regulating intracellular pH.
|
ATP-Dependent Transporters
|
Na+,K+-ATPase, also called the Na+,K+ pump or just the Na+ pump, is found in all cells and is responsible for establishing the cellular gradients for Na+ and K+. These gradients in turn provide energy for several essential cell functions (see Chapter 2). Na+,K+-ATPase is composed of three subunits (α, β, and γ), and the protein exists in the membrane with a stoichiometry of 1α, 1β, 1γ. There are four isoforms of the α subunit and three of the β subunit. The α1 isoform is the most ubiquitous and is expressed in all cells. The α subunit contains binding sites for Na+, K+, and ATP. It is also the subunit that binds cardiac glycosides (e.g., ouabain), which specifically inhibit the enzyme. Although the α subunit is the functional subunit of the enzyme (i.e., it hydrolyzes ATP, binds Na+ and K+, and translocates them across the membrane), it cannot function without the β subunit. The β subunit is responsible for targeting the α subunit to the membrane and also appears to modulate the affinity of Na+,K+-ATPase for Na+ and K+. The γ subunit is a member of a family of proteins called FXYD proteins (so named for the FXYD amino acid sequence found in the protein). There are seven members of this family, and many are associated with Na+,K+-ATPase. However, FXYD2 is the isoform referred to as the γ subunit of Na+,K+-ATPase. FXYD2 is a small protein (61 amino acids in length) that spans the plasma membrane once. It appears to play a role modulating the affinity of Na+,K+-ATPase for Na+, K+, and ATP. |
 |
ATP-dependent transporters, as their name implies, use the energy in ATP to drive the movement of molecules/ions across the membrane. There are two groups of ATP-dependent transporters: ATPase ion transporters and ATP-binding cassette (ABC) transporters. ATPase ion transporters are subdivided into P-type and V-type ATPases.* P-type ATPases share the
feature of being phosphorylated during the transport cycle. Na+,K+-ATPase is an important example of a P-type ATPase. With the hydrolysis of each ATP molecule, 3 Na+ ions are transported out of the cell and 2 K+ ions into the cell. Na+,K+-ATPase is present in all cells and plays a critical role in establishing cellular ion and electrical gradients, as well as maintaining cell volume (see Chapter 2).
|
V-type H+-ATPase is found in the membranes of several intracellular organelles (e.g., endosomes, lysosomes) and as a result is also referred to as vacuolar H+-ATPase. The H+-ATPase on the plasma membrane plays an important role in urinary acidification (see Chapter 36).
|
ABC transporters represent a large group of membrane transporters. They are found in both prokaryotic and eukaryotic cells, and they share a common feature of having amino acid domains that bind ATP (i.e., ATP-binding cassette). There are seven subgroups of ABC transporters in humans and more than 40 specific transporters have been identified. They transport a diverse group of molecules/ions, including Cl-, cholesterol, bile acids, drugs, iron, and organic anions.
|
Cystic fibrosis is an autosomal recessive disease characterized by chronic lung infections, pancreatic insufficiency, and infertility in males. Death usually occurs because of respiratory failure. It is most prevalent in the white population, occurring in 1 in 3000 live births, and is the most common lethal genetic disease in this population. It is a result of mutations in a gene on chromosome 7 that codes for an ABC transporter. To date, more than 1000 mutations in the gene have been identified. The most common mutation is deletion of a phenylalanine at position 508 (ΔF508). This deletion results in defective processing of the protein by the endoplasmic reticulum, and as a result the transporter does not reach the plasma membrane. This transporter, called the cystic fibrosis transmembrane regulator (CFTR), normally functions as a Cl- channel and also regulates other membrane transporters (e.g., the epithelial Na+ channel [ENaC]). Thus, epithelial transport in individuals with cystic fibrosis is defective, which underlies the problems that these patients have. For example, in a normal lung the epithelial cells that line the airway are covered with a layer of mucus that entraps inhaled particulate matter and bacteria. Cilia on the epithelial cells then transport the entrapped material out of the lung, a process termed mucociliary transport (see Chapter 20 for more details). In patients with cystic fibrosis the defective epithelial transport results in thickening of airway mucus, and as a result the cilia cannot transport the entrapped material out of the lung. This in turn leads to recurrent and chronic lung infections. The inflammatory process that accompanies these infections ultimately destroys the lung tissue and causes respiratory failure and death. |
 |
page 9 |  | page 10 |
The plasma membrane of cells is constantly turning over. As a result, membrane proteins are continuously being replaced. One mechanism by which membrane proteins are "tagged" for replacement is by the attachment of ubiquitin to the cytoplasmic portion of the protein. Ubiquitin is a 76-amino acid protein that is covalently attached to the membrane protein (usually to lysine) by a class of enzymes called ubiquitin protein ligases. One important group of these ligases is the Nedd4/Nedd4-like family. Once a membrane protein is ubiquitinated, it undergoes endocytosis and is degraded either by lysosomes or by proteasomes. Cells also contain deubiquitinating enzymes called DUBs. Thus, the amount of protein in a cell depends on the rate that ubiquitin groups are added by ligases versus the rate that they are removed by DUBs. Ubiquitination of plasma proteins provides one mechanism for regulation of membrane transport by the cell. For example, Na+ reabsorption by the distal nephron of the kidney is stimulated by the adrenal hormone aldosterone (see Chapters 33 and 34). One of the actions of aldosterone is to inhibit Nedd4-2. This prevents ubiquitination of the Na+ channel (ENaC) in the apical membrane of epithelial cells in this portion of the nephron. Thus, they are retained for a longer period in the membrane, and as a result more Na+ enters the cell and is thereby reabsorbed by the nephron. |
 |
Table 1-3 is a partial listing of membrane transport proteins that have been well studied and for which much is known about their function (see Fig. 1-5 for some molecular models of membrane transport proteins). Many of these transporters will be considered in greater detail in other chapters.
|
Solute and water can be brought into the cell by the process of endocytosis and released from the cell by the process of exocytosis. In both processes the integrity of the plasma membrane is maintained, and the vesicles that are formed allow transfer of the contents between cellular compartments. In some cells (e.g., the epithelial cells lining the gastrointestinal tract), endocytosis across one membrane of the cell is followed by exocytosis across the opposite membrane. This allows the transport of substances across the epithelium, a process termed transcytosis.
|
Table 1-3.
Examples of Plasma Membrane Transporters |
Water Channels |
Aquaporin (AQP-multiple isoforms) |
Ion Channels |
Na+ K+ Ca++ Cl- Anion Cation | → | Multiple channels exist for each ion listed. They are distinguished by their selectivity, conductance, and mode of regulation (i.e., gating) |
Solute Carriers |
Uniport |
Glucose (GLUT2) |
Fructose (GLUT5) |
Urea (UT-A1) |
Fe+++ (ferroportin/IREG-1) |
Symport |
1Na+-glucose (SGLT2) |
2Na+-glucose (SGLT1) |
Na+-amino acid (multiple transporters) |
Na+-Cl- (NCC/TSC) |
1Na+, 1K+, 2Cl- (NKCC2) |
Na+-3HCO3- (NBC1) |
3Na+-Pi (type IIa phosphate transporter) |
2Na+-1I- (NIS) |
Na+-bile acid (NTCP-multiple isoforms) |
3Na+-dicarboxylate (SDCT-multiple isoforms) |
H+-oligopeptide (PepT and PHT-multiple isoforms) |
H+-Fe+++ (DCT-1) |
K+-Cl- (KCC-multiple isoforms) |
Antiport |
Na+-H+ (NHE-multiple isoforms) |
Cl--HCO3- (AE-1/band three and pendrin) |
3Na+-Ca++ (NCX-multiple isoforms) |
Organic anions (OAT-multiple transporters for different anions) |
Organic cations (OCT and OCTN-multiple isoforms) |
Transport ATPases |
P-Type |
Na+, K+-ATPase |
H+, K+-ATPase |
H+, Ca++-ATPase (PMCA) |
V-Type |
H+-ATPase |
ABC Transporters |
Cystic fibrosis transmembrane regulator (CFTR) |
Multidrug resistance protein (MRP-1) |
Organic anion (MRP-2) |
page 10 |  | page 11 |
Figure 1-5 Molecular models of several membrane transport proteins. |
Endocytosis can be subdivided into three mechanisms. The first is pinocytosis, which consists of the nonspecific uptake of small molecules and water into the cell. Pinocytosis is a prominent feature of the endothelial cells that line capillaries and is responsible for a portion of the fluid exchange that occurs across blood vessels. A second form of endocytosis allows the internalization of large particles (e.g., bacteria,
cell debris). This process is termed phagocytosis and is an important characteristic of cells in the immune system (e.g., neutrophils and macrophages). Often but not always, phagocytosis is a receptor-mediated process. For example, macrophages have receptors on their surface that bind the Fc portion of immunoglobulins. When bacteria invade the body, they are often coated with antibody, a process called opsonization. These bacteria then attach to the membrane of macrophages via the Fc portion of the immunoglobulin and are phagocytosed and destroyed inside the cell. The third mechanism is receptor-mediated endocytosis, which allows the uptake of specific molecules into the cell. In this form of endocytosis, molecules bind to specific receptors on the surface of the
cell. Endocytosis involves a number of accessory proteins, including adaptin, clathrin, and the GTPase dynamin (Fig. 1-6).
|
page 11 |  | page 12 |
Figure 1-6 Receptor-mediated endocytosis. A receptor on the surface of the cell binds the ligand. A clathrin-coated pit is formed, with adaptin linking the receptor molecules to the clathrin. Dynamin, a GTPase, assists in separation of the endocytic vesicle from the membrane. Once inside the cell, the clathrin and adaptin molecules dissociate and are recycled. The uncoated vesicle is then ready to fuse with other organelles in the cell (e.g., lysosome). (Adapted from Ross MH, Pawlina W: Histology, 5th ed. Baltimore, Lippincott Williams & Wilkins, 2006.) |
Cholesterol is an important component of cells (e.g., it is a key component of membranes). However, most cells are unable to synthesize cholesterol and must therefore obtain it from blood. Normally, cholesterol is ingested in the diet and transported via blood in association with lipoproteins. Low-density lipoproteins (LDLs) in blood carry cholesterol to cells, where they bind to LDL receptors on the surface. Once the receptors bind LDL, they collect into "coated pits" and are endocytosed as clathrin-coated vesicles. The endo-some that is formed by the process removes the LDL and recycles the receptor back to the cell surface. The LDL is then degraded in lysosomes and the cholesterol made available to the cell. Defects in the LDL receptor prevent cellular uptake of LDL. Individuals with this defect have elevated levels of blood LDL, often called "bad cholesterol" because it is associated with the development of cholesterol-containing plaques in the smooth muscle layer of arteries. This process, termed atherosclerosis, is associated with an increased risk for heart attacks because of occlusion of the coronary arteries. |
 |
Exocytosis can be either constitutive or regulated. Constitutive secretion is seen, for example, in plasma cells that are secreting immunoglobulin or in fibroblasts secreting collagen. Regulated secretion occurs in endocrine cells, neurons, and exocrine glandular cells (pancreatic acinar cells). In these cells the secretory product (e.g., hormone, neurotransmitter, or
digestive enzyme), after synthesis and processing in the rER and Golgi apparatus, is stored in the cytoplasm in secretory granules until an appropriate signal for secretion is received. These signals may be hormonal or neural. Once the cell receives the appropriate stimulus, the secretory vesicle fuses with the plasma membrane and releases its contents into the extracellular fluid. Fusion of the vesicle with the membrane is mediated by a number of accessory proteins. One important group is the SNAREs. These membrane proteins help target the secretory vesicle to the plasma membrane. The process of secretion is usually triggered by an increase in intracellular [Ca++]. However, two notable exceptions to this general rule exist: renin secretion by juxtaglomerular cells of the kidney is triggered by a decrease in intracellular Ca++ (see Chapters 33 and 34), as is the secretion of parathyroid hormone (PTH) by the parathyroid gland (see Chapter 39).
|
Physiology of Solute and Water Transport
|
As already noted, the plasma membrane, with its hydrophobic core, is an effective barrier to the movement of virtually all biologically important molecules into or out of the cell. Thus, membrane transport proteins provide the pathway that allows transport to occur. However, the presence of a pathway is not sufficient for transport to occur; an appropriate driving force is also required.
|
Diffusion is the process by which molecules move spontaneously from an area of high concentration to one of low concentration. Thus, wherever a concentration gradient exists, diffusion of molecules from the region of high concentration to the region of low concentration will dissipate the gradient (as discussed later, establishment of concentration gradients for molecules requires the expenditure of energy). Diffusion is a random process driven by the thermal motion of the molecules. The rate at which a molecule diffuses from point A to point B is quantified by Fick's first law of diffusion:
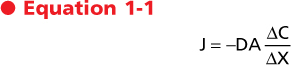 where
- J = flux or rate of diffusion per unit time
- D = diffusion coefficient
- A = area across which the diffusion is occurring
- ΔC = concentration gradient
- ΔX = distance along which the diffusion is occurring
|
page 12 |  | page 13 |
The diffusion coefficient takes into account the thermal energy of the molecule, its size, and the viscosity of the medium through which the diffusion is taking place. For spherical molecules, D is approximated by the Stokes-Einstein equation:
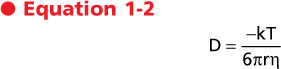 where
- k = Boltzmann's constant
- T = temperature in degrees Kelvin
- r = radius of the molecule
- η = viscosity of the medium
|
By inspection of Equations 1-1 and 1-2, it is evident that the rate of diffusion will be faster for small molecules than for large molecules. In addition, diffusion rates are high at elevated temperatures, in the presence of large concentration gradients, and when occurring in a low-viscosity medium. Holding all other variables constant, the rate of diffusion is linearly related to the concentration gradient.
|
The Fick equation can also be applied to the diffusion of molecules across the plasma membrane. When applied to transport across a membrane, the diffusion coefficient (D) now incorporates the properties of the membrane and especially the ability of the molecule to diffuse through the membrane (i.e., the partition coefficient [β] of the molecule into the membrane). In general, the more lipid soluble the molecule, the larger the partition coefficient and thus the diffusion coefficient, and therefore the rate of diffusion is greater. In this situation, ΔC now represents the concentration gradient across the membrane, A is the membrane area, and ΔX is the thickness of the membrane.
|
A more useful equation for quantitating the diffusion of molecules across the membrane is
 where
- J = flux or rate of diffusion across the membrane
- P = permeability coefficient
- Ci = concentration of the molecule inside the cell
- Co = concentration of the molecule outside the cell
|
This equation is derived from the Fick equation, and P incorporates D, ΔX, and A. P has units of velocity (e.g., cm/sec) and C has the unit mol/cm3. Thus, the unit of flux is mol/cm2/sec. Values of P can be obtained experimentally for any molecule and membrane.
|
As noted, the plasma membrane is an effective barrier to many biologically important molecules. Consequently, diffusion through the lipid phase of the plasma membrane is not an efficient process for movement of these molecules across the membrane. It has been estimated that for a cell 20 μm in diameter with a plasma membrane composed only of phospholipids, dissipation of a urea gradient imposed across the membrane would take about 8 minutes to occur. Similar gradients for glucose and amino acids would take approximately 14 hours to dissipate, whereas ion gradients would take years to dissipate.
|
The term diffusion is often used to describe the movement of some molecules across the cell membrane. However, it is clear that most biologically important molecules cross the membrane via specific membrane transport proteins (e.g., ion channels and solute carriers) and not by simple diffusion through the membrane. Despite the limitations of using diffusion to describe and understand the transport of many molecules across cell membranes, it is important for comprehending the exchange of gases across the airways of the lung (see Chapter 23), the movement of molecules between cells in the extracellular fluid, and the movement of molecules through the cytoplasm of the cell. For example, one of the physiological responses of skeletal muscle to exercise is the recruitment or opening of capillaries that are not patent at rest. This opening of previously closed capillaries increases capillary density and thereby reduces the diffusion distance between the capillary and the muscle fiber so that O2 and cellular fuels (e.g., fatty acids and glucose) can be delivered more quickly to the contracting muscle fiber. It has been estimated that in resting muscle the average distance of a muscle fiber from a capillary is 40 μm. However, with exercise this distance decreases to 20 μm or less.
|
The electrochemical gradient (also called the electrochemical potential difference) is used to quantitate the driving force acting on a molecule to cause it to move across a membrane. The electrochemical gradient for any molecule (Δμx) is calculated as
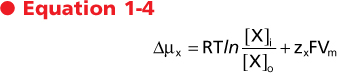 where
- R = gas constant
- T = temperature in degrees Kelvin
- ln = natural logarithm
- [X]i = concentration of X inside the cell
- [X]o = concentration of X outside the cell
- zx = valence of charged molecules
- F = Faraday constant
- Vm = membrane potential
|
page 13 |  | page 14 |
Figure 1-7 Electrochemical gradients and cellular transport of molecules. A, Because glucose is uncharged, the electrochemical gradient is determined solely by the concentration gradient for glucose across the cell membrane. As shown, the glucose concentration gradient would be expected to drive glucose into the cell. B, Because K+ is charged, the electrochemical gradient is determined by both the concentration gradient and the membrane voltage (Vm). The energy in the concentration gradient, determined from the Nernst equation, is 90.8 mV (driving K+ out of the cell). The membrane voltage of -60 mV will drive K+ into the cell. The electrochemical gradient, or the net driving force, is 30.8 mV, which will drive K+out of the cell. |
The electrochemical gradient is a measure of the free energy available to carry out the useful work of transporting the molecule across the membrane. As can be seen, it has two components. One component represents the energy in the concentration gradient for X across the membrane (chemical potential difference). The second component (electrical potential difference) represents the energy associated with moving charged molecules (e.g., ions) across the membrane when a membrane potential exits (i.e., Vm ≠ 0 mV). Thus, for movement of glucose across a membrane, only the concentrations of glucose inside and outside the cell need be considered. However, movement
of K+ across the membrane, for example, would be determined by both the concentration of K+ inside and outside the cell and the membrane voltage (Fig. 1-7).
|
Equation 1-4 can be used to derive the Nernst equation by considering the situation in which the molecule is at equilibrium across the membrane (i.e., Δμ = 0).
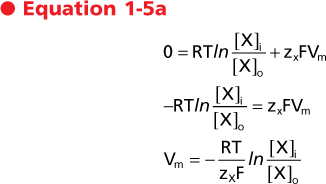 Alternatively
|
The value of Vm calculated by the Nernst equation represents the equilibrium condition and is referred to as the Nernst equilibrium potential (Ex). It should be apparent that the Nernst equilibrium potential quantitates the energy in a concentration gradient and expresses that energy in millivolts. For example, for the cell depicted in Figure 1-7, B, the energy in the K+ gradient (EK) is 90.8 mV (which causes K+ to move out of the cell). This is opposite and of greater magnitude than the energy in the membrane voltage (Vm = -60 mV), which will cause K+ to enter the cell. As a result, the electrochemical gradient is such that the net movement of K+ across the membrane will be out of the cell. Another way to state this is that the net driving force for K+ (Vm - EK) is 30.8 mV (which drives K+ out of the cell).
|
At body temperature (37°C) and by replacing the natural logarithm with a base 10 logarithm, the Nernst equation can be written as follows:
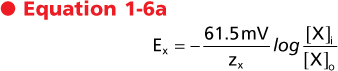 or
|
These are the most common forms of the Nernst equation in use. By inspection of these equations it is apparent that for a univalent ion (e.g., Na+, K+, Cl-), a 10-fold concentration gradient across the membrane is equivalent in energy to an electrical potential difference of 61.5 mV and a 100-fold gradient is equivalent to 123 mV. Similarly, for a divalent ion (e.g., Ca++), a 10-fold concentration gradient is equivalent to a 30.7-mV electrical potential difference because z in the above equations is equal to 2.
|
Active and Passive Transport
|
page 14 |  | page 15 |
When the net movement of a molecule across a membrane occurs in the direction predicted by the electrochemical gradient, the movement is termed passive
transport. Thus, for the examples given in Figure 1-7, movement of glucose into the cell and movement of K+ out of the cell would be considered passive transport. Transport that is passive is sometimes referred to as either "downhill transport" or transport "with the electrochemical gradient." In contrast, if the net movement of a molecule across the membrane is opposite that predicted by the electrochemical gradient, the movement is termed active transport. Active transport is sometimes referred to as either "uphill transport" or transport "against the electrochemical gradient."
|
Figure 1-8 Examples of several membrane transporters illustrating primary active, passive, and secondary active transport. See text for details. |
When considering the various classes of plasma membrane transport proteins, movement of H2O through water channels is a passive process (see later), as is the movement of ions through ion channels and the transport of molecules via uniporters (e.g., transport of glucose via GLUT1). The ATPase-dependent transporters can use the energy in ATP to drive the active transport of molecules (e.g., Na+,K+-ATPase). Because the transport is directly coupled to the hydrolysis of ATP, it is referred to as primary active transport. Solute carriers that couple the movement of two or more molecules will often transport one or more molecules against their respective electrochemical gradient by using the energy in the electrochemical gradient of the other molecule or molecules to drive this transport. When this occurs, the molecule or molecules transported against their electrochemical
gradient are said to be transported by secondary active mechanisms (Fig. 1-8).
|
OSMOSIS AND OSMOTIC PRESSURE
|
Movement of water across cell membranes occurs by the process of osmosis. The movement of water is passive, with the driving force for this movement being the osmotic pressure difference across the cell membrane. Figure 1-9 illustrates the concept of osmosis and measurement of the osmotic pressure of a solution.
|
Osmotic pressure is determined solely by the number of molecules in that solution. It is not dependent on such factors as the size of the molecules, their mass, or their chemical nature (e.g., valence). Osmotic pressure (π), measured in atmospheres (atm), is calculated by van't Hoff's Law as
 where
- n = number of dissociable particles per molecule
- C = total solute concentration
- R = gas constant
- T = temperature in degrees Kelvin
|
page 15 |  | page 16 |
The epithelial cells that line the gastrointestinal tract (small intestine) and make up the proximal tubule of the kidney transport glucose. In the gastrointestinal tract, glucose is absorbed from ingested food. In the kidney, the proximal tubule reabsorbs the glucose that was filtered at the glomerulus and thereby prevents it from being lost in urine. Uptake of glucose into the epithelial cell from the lumen of the small intestine and from the lumen of the proximal tubule is a secondary active process involving the Na+-glucose symporters SGLT1 and SGLT2. SGLT2 transports 1 glucose molecule with 1 Na+ ion, and the energy in the electrochemical gradient for Na+ (into the cell) is used to drive the secondary active uptake of glucose. Using the equation for calculating the electrochemical gradient, as rearranged below, and assuming a membrane potential (Vm) of -60 mV and a 10-fold [Na+] gradient across the membrane, an approximate 100-fold glucose gradient could be generated by SGLT2.
 |
Thus, if the intracellular [glucose] was 2 mmol/L; the cell could lower the luminal [glucose] to approximately 0.02 mmol/L. However, by increasing the number of Na+ ions transported with glucose from 1 to 2, SGLT1 can generate a nearly 10,000-fold glucose gradient.
 |
Again, assuming an intracellular [glucose] of 2 mmol/L, SGLT1 could remove virtually all glucose from either the lumen of the small intestine or the lumen of the proximal tubule (i.e., luminal [glucose] of ≈0.0002mmol/L). |
For a molecule that does not dissociate in water, such as glucose or urea, a solution containing 1 mmol/L of these solutes at 37°C can exert an osmotic pressure of 2.54 × 10-2 atm as calculated by Equation 1-7 with the following values:
- n = 1
- C = 0.001 mol/L
- R = 0.082 atm L/mol °K
- T = 310°K
|
Because 1 atm equals 760 mm Hg at sea level, π for this solution can also be expressed as 19.3 mm Hg. Alternatively, osmotic pressure can be expressed in terms of osmolarity (see the following). Thus, regardless of the type of molecules, a solution containing 1 mmol/L of solute exerts an osmotic pressure of 1 mOsm/L.
|
For molecules that dissociate in a solution, n in Equation 1-7 will have a value other than 1. For example, a 150-mmol/L solution of NaCl has an osmolarity of approximately 300 mOsm/L because each molecule of NaCl dissociates into an Na+ and a Cl- ion (i.e., n = 2).* If dissociation of a molecule into its component ions is not complete, n will not be an integer. Accordingly, the osmolarity of any solution can be calculated as
|
Osmolarity versus Osmolality
|
The terms osmolarity and osmolality are frequently confused and incorrectly interchanged. Osmolarity refers to the osmotic pressure generated by the dissolved solute molecules in 1 L of solvent, whereas osmolality is the number of molecules dissolved in 1 kg of solvent. For dilute solutions, the difference between osmolarity and osmolality is insignificant. Measurements of osmolarity are dependent on temperature because the volume of solvent varies with temperature (i.e., the volume is larger at higher temperatures). In contrast, osmolality, which is based on the mass of the solvent, is independent of temperature. For this reason, osmolality is the preferred term for biological systems and is used throughout this book. Osmolality has the units of Osm/kg H2O. Because of the dilute nature of physiological solutions and because water is the solvent, osmolality is expressed as milliosmoles per kilogram water (mOsm/kg H2O).
|
Table 1-4 shows the relationship between molecular weight, equivalence, and osmoles for a number of physiologically significant molecules.
|
The tonicity of a solution is related to the effect of the solution on the volume of a cell. Solutions that do not change the volume of a cell are said to be isotonic. A hypotonic solution causes a cell to swell, whereas a hypertonic solution causes a cell to shrink. Though related to osmolality, tonicity also takes into consideration the ability of the molecules in solution to cross the cell membrane.
|
page 16 |  | page 17 |
|
Figure 1-9 Schematic representation of osmotic water movement and the generation of osmotic pressure. Compartment A and compartment B are separated by a semipermeable membrane (i.e., the membrane is highly permeable to water, but impermeable to solute). Compartment A contains a solute, whereas compartment B contains only distilled water. Over time, water will move by osmosis from compartment B to compartment A. (Note: This water movement is driven by the concentration gradient for water. Because of the presence of solute particles in compartment A, the concentration of water in compartment A is less than that in compartment B. Consequently, water moves across the semipermeable membrane from compartment B to compartment A down its gradient.) This will raise the level of fluid in compartment A and decrease the level in compartment B. At equilibrium, the hydrostatic pressure exerted by the column of water (h) will stop the movement of water from compartment B to compartment A. This pressure will be opposite and equal to the osmotic pressure exerted by the solute particles in compartment A. (Redrawn from Koeppen BM, Stanton BA: Renal Physiology, 4th ed. St. Louis, Mosby, 2006.) |
Table 1-4.
Units of Measurement for Physiologically Significant Substances |
Substance | Atomic/Molecular Weight | Equivalents/mol | Osmoles/mol |
Na+ | 23.0 | 1 | 1 |
K+ | 39.1 | 1 | 1 |
Cl- | 35.4 | 1 | 1 |
HCO3- | 61.0 | 1 | 1 |
Ca++ | 40.1 | 2 | 1 |
Phosphate (Pi) | 95.0 | 3 | 1 |
NH4+ | 18.0 | 1 | 1 |
NaCl | 58.4 | 2* | 2 |
CaCl2 | 111 | 4 | 3 |
Glucose | 180 | | 1 |
Urea | 60 | | 1 |
*One equivalent each from Na+ and Cl-. NaCl does not dissociate completely in solution. The actual osmoles/mol is 1.88. However, for simplicity, a value of 2 is often used. Ca++ contributes two equivalents, as do the 2Cl- ions.
|
Consider two solutions: a 300-mmol/L solution of sucrose and a 300-mmol/L solution of urea. Both solutions have an osmolality of 300 mOsm/kg H2O and are therefore said to be isosmotic (i.e., they have the same osmolality). When red blood cells, which for the purpose of this example also have an intracellular fluid osmolality of 300 mOsm/kg H2O, are placed in the two solutions, those in the sucrose solution maintain their normal volume, whereas those placed in urea swell and eventually burst. Thus, the sucrose solution is isotonic and the urea solution is hypotonic. The differential effect of these solutions on red cell volume is related to the permeability of the plasma membrane to sucrose and urea. The red cell membrane contains uniporters for urea. Thus, urea easily crosses the cell membrane (i.e., urea is permeable), driven by the concentration
gradient (i.e., extracellular [urea] > intracellular [urea]). In contrast, the red cell membrane does not contain sucrose transporters, and sucrose cannot enter the cell (i.e., sucrose is impermeable).
|
To exert osmotic pressure across a membrane, a molecule must not cross the membrane. Because the red cell membrane is impermeable to sucrose, it exerts an osmotic pressure opposite and equal to the osmotic pressure generated by the contents of the red cell (in this case 300 mOsm/kg H2O). In contrast, urea is readily able to cross the red blood cell membrane, and it cannot exert an osmotic pressure to balance that generated by the intracellular solutes of the red blood cell. Consequently, sucrose is termed an effective osmole, whereas urea is an ineffective osmole.
|
To take into account the effect of a molecule's membrane permeability on osmotic pressure, it is necessary to rewrite Equation 1-7 as
 where σ is the reflection coefficient or osmotic coefficient and π is a measure of the relative ability of the molecule to cross the cell membrane.
|
page 17 |  | page 18 |
Figure 1-10 Relationship between the concentration of plasma proteins in solution and the osmotic pressure (oncotic pressure) that they generate. Protein concentration is expressed as g/dl. Normal plasma protein concentration is indicated. Note how the actual pressure generated exceeds that predicted by van't Hoff's law. (Redrawn from Koeppen BM, Stanton BA: Renal Physiology, 4th ed. St. Louis, Mosby, 2006.) |
For a molecule that can freely cross the cell membrane, such as urea in the aforementioned example, σ = 0, and no effective osmotic pressure is exerted (i.e., urea is an ineffective osmole for red blood cells). In contrast, σ = 1 for a solute that cannot cross the cell membrane (i.e., sucrose). Such a substance is said to
be an effective osmole. Many molecules are neither completely able nor completely unable to cross cell membranes (i.e., 0 < σ < 1), and they generate an osmotic pressure that is only a fraction of what is expected from the molecule's concentration in solution.
|
Oncotic pressure is the osmotic pressure generated by large molecules (especially proteins) in solution. As illustrated in Figure 1-10, the magnitude of the osmotic pressure generated by a solution of protein does not conform to van't Hoff's law. The cause of this anomalous relationship between protein concentration and osmotic pressure is not completely understood but appears to be related to the size and shape of the protein molecule. For example, the correlation to van't Hoff's law is more precise with small, globular proteins than with larger protein molecules.
|
The oncotic pressure exerted by proteins in human plasma has a normal value of approximately 26 to 28 mm Hg. Although this pressure appears to be small when considered in terms of osmotic pressure (28 mm Hg ≈ 1.4 mOsm/kg H2O), it is an important force involved in fluid movement across capillaries (see Chapter 17).
|
The specific gravity of urine is sometimes measured in clinical settings and used to assess the urine-concentrating ability of the kidney. The specific gravity of urine varies in proportion to its osmolality. However, because specific gravity depends on both the number of molecules and their weight, the relationship between specific gravity and osmolality is not always predictable. For example, patients who have been injected with radiocontrast dye (molecular weight >500g/mol) for x-ray studies can have high values of urine specific gravity (1.040 to 1.050) even though urine osmolality is similar to that of plasma (e.g., 300 mOsm/kg H2O). |
 |
The total concentration of all molecules in a solution can also be measured as specific gravity. Specific gravity is defined as the weight of a volume of solution divided by the weight of an equal volume of distilled water. Thus, the specific gravity of distilled water is 1. Because biological fluids contain a number of different molecules, their specific gravities are greater than 1. For example, normal human plasma has a specific gravity in the range of 1.008 to 1.010.
|
page 18 |  | page 19 |
- The plasma membrane is a lipid bilayer composed of phospholipids and cholesterol into which a wide range of proteins are embedded. One class of these membrane proteins (membrane transport proteins or transporters) is involved in the selective and regulated transport of molecules into and out of the cell. These transporters include water channels (aquaporins), ion channels, solute carriers, and ATP-dependent transporters.
- Movement of molecules across the plasma membrane through ion channels via solute carriers is driven by chemical concentration gradients and electrical potential differences (charged molecules only). The electrochemical gradient is used to quantitate this driving force. ATP-dependent transporters use the energy in ATP to transport molecules across the membrane and often establish the chemical and electrical gradients that then drive the transport of other molecules through channels, or via solute carriers. Water movement through aquaporins is driven by an osmotic pressure difference across the membrane.
- Transport across the membrane is classified as passive or active. Passive transport describes the movement of molecules as expected from the electrochemical gradient for that molecule. Active
transport represents transport against the electrochemical gradient. Active transport is further divided into primary active and secondary active transport. Primary active describes transport directly coupled to the hydrolysis of ATP (e.g., ATP-dependent transporters). Secondary active transport occurs with coupled solute carriers, where passive movement of one or more molecules drives the active transport of other molecules (e.g., Na+-glucose symporter, Na+-H+ antiporter).
|
 |
|