23 Oxygen and Carbon Dioxide Transport
|
The respiratory and circulatory systems function together to transport sufficient oxygen (O2) from the lungs to the tissues to sustain normal cellular activity and to transport carbon dioxide (CO2) from the tissues to the lungs for expiration. CO2, a product of active cellular glucose metabolism, is transported from the tissues via systemic veins to the lungs, where it is expired (Fig. 23-1). To enhance uptake and transport of these gases between the lungs and tissues, specialized mechanisms (e.g., O2-hemoglobin binding and HCO3- transport of CO2) have evolved that enable O2 uptake and CO2 expiration to occur simultaneously. Moreover, these specialized mechanisms facilitate uptake of O2 and expiration of CO2. To gain an understanding of the mechanisms involved in the transport of these gases, one must consider gas diffusion properties, as well as transport and delivery mechanisms.
|
Gas movement throughout the respiratory system occurs predominantly via diffusion. The respiratory and circulatory systems contain several unique anatomic and physiological features to facilitate gas diffusion: (1) large surface areas for gas exchange (alveolar to capillary and capillary to tissue membrane barriers) with short distances to travel, (2) substantial partial pressure gradient differences, and (3) gases with advantageous diffusion properties. Transport and delivery of O2 from the lungs to the tissue and vice versa for CO2 are dependent on basic gas diffusion laws.
|
Gases in the Lung Diffuse from Regions of Higher to Lower Partial Pressure
|
The process of gas diffusion is passive and similar whether diffusion occurs in a gaseous or liquid state. The rate of diffusion of a gas through a liquid is described by Graham's law, which states that the rate is directly proportional to the solubility coefficient of the gas and inversely proportional to the square root of its molecular weight. Calculation of the diffusion properties for O2 and CO2 reveals that CO2 diffuses approximately 20 times faster than O2 does. Rates of O2 diffusion from the lungs into blood and from blood into tissue, and vice versa for CO2, are predicted by Fick's law of gas diffusion (Fig. 23-2). Fick's law states that the diffusion of a gas (Vgas) across a sheet of tissue is directly related to the surface area (A) of the tissue, the diffusion constant (D) of the specific gas, and the partial pressure difference (P1 - P2) of the gas on each side of the tissue and is inversely related to tissue thickness (T). That is,
|
The ratio A · D/T represents the conductance of a gas from the alveolus to blood. The diffusing capacity of the lung (DL) is its conductance (A · D/T) when considered for the entire lung; thus, applying Fick's equation, DL can be calculated as follows:
|
Fick's law of diffusion could be used to assess the diffusion properties of O2 in the lung, except that ΔP (alveolar - capillary Po2) cannot be determined because capillary Po2 cannot be measured. This limitation can be overcome by using carbon monoxide (CO) rather than O2. Because CO has low solubility in the capillary membrane, the rate of CO equilibrium across the capillary is slow and the partial pressure of CO in capillary blood remains close to zero. This is in contrast to the high solubility of CO in blood. Thus, the only limitation for diffusion of CO is the alveolar-capillary membrane, which makes CO a useful gas for calculating DL. The capillary partial pressure (P2 above) is essentially zero for CO, and therefore DL can be measured from V.co and the average partial pressure of CO in the alveolus. That is,
|
Assessment of DLCO has become a classic measurement of the diffusion barrier of the alveolar-capillary membrane. It is useful in the differential diagnosis of certain restrictive and obstructive lung diseases, such as interstitial pulmonary fibrosis and emphysema.
|
page 459 |  | page 460 |
Figure 23-1 Oxygen and CO2 transport in arterial and venous blood. Oxygen in arterial blood is transferred from arterial capillaries to tissues. The flow rates for O2 and CO2 are shown for 1 L of blood. The ratio of CO2 production to O2 consumption is the respiratory exchange ratio, R, which at rest is approximately 0.80. |
Figure 23-2 Fick's law states that diffusion of a gas across a sheet of tissue is directly related to the surface area of the tissue, the diffusion constant of the specific gas, the partial pressure difference of the gas on each side of the tissue and is inversely related to tissue thickness. |
A patient with interstitial pulmonary fibrosis (a restrictive lung disease) inhales a single breath of 0.3% CO from residual volume to total lung capacity. He holds his breath for 10 seconds and then exhales. After discarding the exhaled gas from the dead space, a representative sample of alveolar gas from late in exhalation is obtained. The average alveolar CO pressure is 0.1 mm Hg, and 0.25 mL of CO has been taken up. The diffusion capacity for CO in this patient is
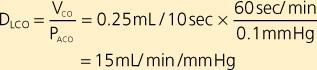 |
The normal range for DLCO is 20 to 30 mL/min/mm Hg. Patients with interstitial pulmonary fibrosis have an initial alveolar inflammatory response with subsequent scar formation within the interstitial space. The inflammation and scar tissue thicken the interstitial space and thus make it more difficult for gas diffusion to occur, which results in decreased DLCO. This is a classic characteristic of certain types of restrictive lung disease; gas readily enters the alveolus but is inhibited in its ability to diffuse into blood. |
Oxygen and Carbon Dioxide Exchange in the Lung Is Perfusion Limited
|
Different gases have different solubility factors. Gases that are insoluble in blood (i.e., anesthetic gases, nitrous oxide and ether) do not chemically combine with proteins in blood and equilibrate rapidly between alveolar gas and blood. The equilibration occurs in less time than the 0.75 second that the red blood cell spends in the capillary bed (capillary transit time). The diffusion of insoluble gases between alveolar gas and blood is considered perfusion limited because the partial pressure of gas in the blood leaving the capillary has reached equilibrium with alveolar gas and is limited only by the amount of blood perfusing the alveolus. In contrast, a diffusion limited gas, such as CO, has low solubility in the alveolar-capillary membrane but high solubility in blood because of its high affinity for hemoglobin (Hgb). These features prevent the equilibration of CO between alveolar gas and blood during the red blood cell transit time.
|
page 460 |  | page 461 |
Figure 23-3 Uptake of nitrous oxide (N2O), CO, and O2 in blood relative to their partial pressures and the transit time of the red blood cell in the capillary. For gases that are perfusion limited (N2O and O2), their partial pressures have equilibrated with alveolar pressure before exiting the capillary. In contrast, the partial pressure of CO, a gas that is diffusion limited, does not reach equilibrium with alveolar pressure. O2 uptake in rare conditions can become diffusion limited. |
The high affinity of CO for Hgb enables large amounts of CO to be taken up in blood with little or no appreciable increase in its partial pressure. Gases that are chemically bound to Hgb do not exert a partial pressure in blood. Like CO, both CO2 and O2 have relatively low solubility in the alveolar-capillary membrane but high solubility in blood because of their ability to bind to Hgb. However, their rate of equilibration is sufficiently rapid for complete equilibration to occur during the transit time of the red blood cell within the capillary. Equilibration for O2 and CO2 usually occurs within 0.25 second. Thus, O2 and CO2 transfer is normally perfusion limited. The partial pressure of a diffusion
limited gas (i.e., CO) does not reach equilibrium with the alveolar pressure over the time that it spends in the capillary (Fig. 23-3). Although CO2 has a greater rate of diffusion in blood than O2 does, it has a lower membrane-blood solubility ratio and consequently takes approximately the same amount of time to reach equilibration in blood.
|
Diffusion limitation for O2 and CO2 would occur if red blood cells spent less than 0.25 second in the capillary bed. Occasionally, this can be seen in very fit athletes during vigorous exercise and in healthy subjects who exercise at high altitude.
|
Oxygen is carried in blood in two forms: dissolved O2 and O2 bound to Hgb. The dissolved form is measured clinically in an arterial blood gas sample as Pao2. Only a small percentage of O2 in blood is in the dissolved form, and its contribution to O2 transport under normal conditions is almost negligible. However, dissolved O2 can become a significant factor in conditions of severe hypoxemia. Binding of O2 to Hgb to form oxyhemoglobin within red blood cells is the primary transport mechanism of O2. Hgb not bound to O2 is referred to as deoxyhemoglobin or reduced Hgb. The O2-carrying capacity of blood is enhanced about 65 times by its ability to bind to Hgb.
|
Hgb is the major transport molecule for O2. The Hgb molecule is a protein with two major components: four nonprotein heme groups each containing iron in the reduced ferrous (Fe+++) form, which is the site of O2 binding, and a globin portion consisting of four polypeptide chains. Normal adults have two α-globin chains and two β-globin chains (HgbA), whereas children younger than 1 year have fetal Hgb (HgbF) consisting of two α chains and two γ chains. This difference in the structure of HgbF increases its affinity for O2 and aids in the transport of O2 across the placenta. In addition, HgbF is not inhibited by 2,3-diphosphoglycerate (2,3-DPG), a product of glycolysis, thus further enhancing O2 uptake.
|
Binding of O2 to Hgb alters the ability of Hgb to absorb light. This effect of O2 on Hgb is responsible for the change in color between oxygenated arterial blood (bright red) and deoxygenated venous blood (dark-red bluish). Binding and dissociation of O2 with Hgb occur in milliseconds, thus facilitating O2 transport because red blood cells spend 0.75 second in the capillaries. There are approximately 280 million Hgb molecules per red blood cell, which provides an efficient mechanism to transport O2. Myoglobin, a protein similar in structure and function to Hgb, has only one subunit of the Hgb molecule. It aids in the transfer of O2 from blood to muscle cells and in the storage of O2, which is especially critical in O2-deprived conditions.
|
Abnormalities of the Hgb molecule occur with mutations in the amino acid sequence (i.e., sickle cell disease) or in the spatial arrangement of the globin polypeptide chains and result in abnormal function. Compounds such as CO, nitrites (nitric oxide [NO]), and cyanides can oxidize the iron molecule in the heme group and change it from the reduced ferrous state to the ferric state (Fe++++), which reduce the ability of O2 to bind to Hgb.
|
The Oxyhemoglobin Dissociation Curve
|
page 461 |  | page 462 |
In an inherited homozygous condition known as sickle cell disease, individuals have an amino acid substitution (valine for glutamic acid) on the β chain of the Hgb molecule. This creates a sickle cell Hgb (HgbS), which when not bound to oxygen (deoxyhemoglobin or desaturated Hgb), can transform into a gelatinous material that distorts the normal biconcave shape of the red blood cell to a crescent or "sickle" form. This change in shape increases the tendency of the red blood cell to form thrombi or clots that obstruct small vessels and creates a clinical condition known as "acute sickle cell episode." The symptoms of such an episode vary depending on the site of the obstruction (i.e., stroke, pulmonary infarction) but are commonly associated with intense pain. The spleen is a common site of infarction, and the ensuing tissue damage compromises the immune capabilities of individuals and renders them susceptible to recurrent infections. In the homozygous form this is a life-shortening condition; however, in the heterozygous form, individuals are resistant to malaria. Thus, there is a survival advantage to a heterozygous individual in regions of the world where malaria is prevalent, which may explain why the sickle cell mutation has been preserved through evolution. The increased affinity of HgbF for O2 renders advantages to individuals with sickle cell disease in that the cells do not desaturate as much when O2 is released from Hgb to the tissue and thus are less likely to sickle. Sickle cell disease is most prevalent in individuals of African American descent but is also observed in Hispanic, Turkish, Asian, and other ethnic groups. |
 |
In the alveoli the majority of O2 in plasma quickly diffuses into red blood cells and chemically binds to Hgb. This process is reversible such that Hgb gives up its O2 to the tissue. The oxyhemoglobin dissociation curve illustrates the relationship between Po2 in blood and the number of O2 molecules bound to Hgb (Fig. 23-4). The S shape of the curve demonstrates the dependence of Hgb saturation on Po2, especially at partial pressures lower than 60 mm Hg. The clinical significance of the flat portion of the oxyhemoglobin dissociation curve (>60 mm Hg) is that a drop in Po2 over a wide range of partial pressures (100 to 60 mm Hg) has only minimal effect on Hgb saturation, which remains at 90% to 100%, a level sufficient for normal O2 transport and delivery. The clinical significance of the steep portion (<60 mm Hg) of the curve is that a large amount of O2 is released from Hgb with only a small change in Po2, which facilitates the release and diffusion of O2
into tissue. The point on the curve where 50% of Hgb is saturated with O2 is called the P50, and it is 27 mm Hg in normal adults (Fig. 23-4).
|
Physiological Factors That Shift the Oxyhemoglobin Dissociation Curve
|
The oxyhemoglobin dissociation curve can be shifted in numerous clinical conditions either to the right or to the left (Fig. 23-5). The curve is shifted to the right when the affinity of Hgb for O2 decreases, which enhances O2 dissociation. This results in decreased Hgb binding to O2 at a given Po2, thus increasing the P50. When the affinity of Hgb for O2 increases, the curve is shifted to the left, which reduces the P50. In this state, O2 dissociation and delivery to tissue are inhibited. Shifts to the right or left of the dissociation curve have little effect when they occur at O2 partial pressures within the normal range (80 to 100 mm Hg). However, at O2 partial pressures below 60 mm Hg (steep part of the curve), shifts in the oxyhemoglobin dissociation curve can dramatically influence O2 transport.
|
Figure 23-4 Oxyhemoglobin dissociation curve showing the relationship between the partial pressure of O2 in blood and the percentage of Hgb binding sites that are occupied by oxygen molecules (percent saturation). Adult hemoglobin (HgbA) is about 50% saturated at a Po2 of 27 mm Hg, 90% saturated at 60 mm Hg, and about 98% saturated at 100 mm Hg. The P50 is the partial pressure at which Hgb is 50% saturated with O2. When the O2 dissociation curve shifts to the right, P50 increases. When the curve shifts to the left, P50 decreases. |
Figure 23-5 Factors that shift the oxyhemoglobin dissociation curve. |
page 462 |  | page 463 |
Changes in blood pH shift the oxyhemoglobin dissociation curve. An increase in CO2 production by tissue
and release into blood results in the generation of hydrogen ions (H+) and a decrease in pH. This shifts the dissociation curve to the right, which has a beneficial effect by aiding in the release of O2 from Hgb for diffusion into tissues. The shift to the right in the dissociation curve is due to the decrease in pH and to a direct effect of CO2 on Hgb. This effect of CO2 on the affinity of Hgb for O2 is known as the Bohr effect, and
it serves to enhance O2 uptake in the lungs and delivery of O2 to tissues. Conversely, as blood passes through the lungs, CO2 is exhaled, thereby resulting in an increase in pH, which causes a shift to the left in the oxyhemoglobin dissociation curve. Increased body temperature, such as during exercise, shifts the oxyhemoglobin dissociation curve to the right and enables more O2 to be released to tissues where it is needed because the demand increases. During cold weather, a decrease in body temperature, especially in the extremities (lips, fingers, toes, and ears), shifts the O2 dissociation curve to the left (higher Hgb affinity). In this instance Pao2 may be normal, but release of O2 in these extremities is not facilitated. That is why these anatomic areas display a bluish coloration with exposure to cold.
|
Mature red blood cells do not have mitochondria, and therefore they respire via anaerobic glycolysis. Large quantities of a metabolic intermediary, 2,3-DPG, are formed in the red blood cell during glycolysis, and the affinity of Hgb for O2 decreases as 2,3-DPG levels increase. Thus, the oxyhemoglobin dissociation curve shifts to the right. Although the binding sites of 2,3-DPG and O2 differ on the Hgb molecule, binding of 2,3-DPG creates an allosteric effect that inhibits the binding of O2. Conditions that increase 2,3-DPG include hypoxia, decreased Hgb, and increased pH. Decreased levels of 2,3-DPG are observed in stored blood samples and thus may present a problem to transfusion recipients because of the greater affinity of Hgb for O2, which inhibits the unloading of O2 to tissues.
|
As discussed previously, fetal Hgb has a greater affinity for O2 than adult Hgb does. Fetal Hgb thus shifts the oxyhemoglobin dissociation curve to the left.
|
Figure 23-6 Oxyhemoglobin and carboxyhemoglobin dissociation curves. |
Carbon monoxide (CO) binds to the heme group of the Hgb molecule at the same site as O2 and forms carboxyhemoglobin (HgbCO). A major difference between the ability of CO versus CO2 to bind to Hgb is illustrated by comparing the oxyhemoglobin and carboxyhemoglobin dissociation curves. The affinity of CO for Hgb is about 200 times greater than it is for O2 (Fig. 23-6). Thus, small amounts of CO can greatly influence the binding of O2 to Hgb. In the presence of CO, the affinity of Hgb for O2 is enhanced. This shifts the dissociation curve to the left, which further prevents the unloading and delivery of O2 to tissues. As the Pco2 of blood approaches 1.0 mm Hg, all of the Hgb binding sites are occupied by CO, and Hgb is unable to bind to O2. This situation is not compatible with life and is the cause of death in individuals with CO poisoning. In healthy individuals, HgbCO occupies about 1% to 2% of the Hgb binding sites; however, in cigarette smokers and in individuals who reside in high-density urban traffic areas, occupation of Hgb binding sites can be increased to 10%. Levels above 5% to 7% are considered hazardous. Treatment of individuals with high levels of CO, such as after inhaling car exhaust or
inhaling smoke from a burning building, consists of administering high concentrations of O2 to displace CO from Hgb. Increasing the ambient pressure above atmospheric pressure, through the use of a barometric chamber, substantially increases the O2 tension, which promotes the dissociation of CO from Hgb. Another gas, NO, has great affinity (200,000 times greater than O2) for Hgb, and it binds irreversibly to Hgb at the same site as O2 does. Endothelial cells synthesize NO, which has vasodilation properties. Thus, NO is used therapeutically as an inhalant in patients with pulmonary hypertension to reduce pressure. Although NO poisoning is not common, one should be cautious when administering NO therapy for long periods. Hgb-bound CO and NO is referred to as methemoglobin. Under normal conditions, about 1% to 2% of Hgb is bound to CO and NO.
|
Oxygen Saturation, Content, and Delivery
|
Each Hgb molecule can bind up to four O2 atoms, and each gram of Hgb can bind up to 1.34 mL of O2. The term O2 saturation (So2) refers to the amount of O2 bound to Hgb relative to the maximal amount of O2 (100% O2 capacity) that can bind Hgb. At 100% O2 capacity, the heme groups of the Hgb molecules are fully saturated with O2, and at 75% So2, three of the four heme groups are occupied. Binding of O2 to each heme group increases the affinity of the Hgb molecule to bind additional O2. The O2 content in blood is the sum of the O2 bound to Hgb and the dissolved O2. Oxygen content is decreased in the presence of increased CO2 and CO and in individuals with anemia (Fig. 23-7).
|
page 463 |  | page 464 |
Table 23-1.
Tissue Hypoxia |
Type of Hypoxia | Cause | PaO2 | CaO2 | Amount of O2 Delivered | Amount of O2 Used |
Hypoxic | Pulmonary disease with ↓ PaO2 ↓ V/Q ratio | Low | Low | Low | Normal |
Circulatory | Vascular disease Arteriovenous shunt | Normal | Normal | Low | Normal |
Anemic | CO poisoning Anemia | Normal | Low | Normal | Normal |
Histotoxic | Cyanide poisoning Sodium azide | Normal | Normal | Normal | Low |
Figure 23-7 A comparison of O2 content curves under three conditions shows why HgbCO dramatically reduces the O2 transport system. Fifty percent HgbCO represents binding of half the circulating Hgb with CO. The 50% Hgb and 50% HgbCO curves show the same decreased O2 content in arterial blood. However, CO has a profound effect in lowering venous Po2. The arterial (a) and mixed venous (v) points of constant cardiac output are indicated. |
Oxygen delivery from the lungs to tissues is dependent on several factors, including cardiac output, the Hgb content of blood, and the ability of the lung to oxygenate the blood. Not all of the O2 carried in blood is unloaded at the tissue level. The actual O2 extracted from blood by the tissue is the difference between the arterial O2 content and the venous O2 content times
cardiac output. Under normal conditions, Hgb leaves the lung 75% saturated with O2, but only about 25% is actually used by tissues. Hypothermia, relaxation of skeletal muscles, and an increase in cardiac output reduce O2 consumption. Conversely, a decrease in cardiac output, anemia, hyperthermia, and exercise increase O2 consumption.
|
Tissue hypoxia refers to a condition in which insufficient O2 is available to cells to maintain adequate aerobic metabolism. Thus, anaerobic metabolism is stimulated and results in the generation of increased levels of lactate and H+ and the subsequent formation of lactic acid. The net result can lead to a significant decrease in blood pH. In cases of severe hypoxia, the extremities, toes, and fingertips may appear blue-gray (cyanotic) because of lack of O2 and increased deoxyhemoglobin. There are four major types of tissue hypoxia (Table 23-1) that can occur via different mechanisms, the most common being hypoxic hypoxia. Hypoxic hypoxia is caused by a variety of lung diseases (e.g., chronic obstructive pulmonary disease, pulmonary fibrosis, neuromuscular diseases) that result in decreased Pao2 or Cao2, or both, with a subsequent decrease in the delivery of O2 to tissues. Circulatory (stagnate) hypoxia is caused by reduced blood flow to an organ and is usually due to a vascular disease or an arteriovenous shunt. Anemic hypoxia is caused by an inability of blood to carry sufficient O2 because of either low Hgb (anemia) or an inability of Hgb to carry O2 (as in the case of CO poisoning). Histotoxic hypoxia is often caused by poisons (i.e., cyanide, sodium azide, and some pesticides) that block the electron transport system in mitochondria and prevent the utilization of O2 by the cell.
|
Tissue oxygenation depends on the concentration of Hgb and thus on the number of red blood cells available in the circulation. Red blood cell production (erythropoiesis) in the bone marrow is controlled by the hormone erythropoietin, which is synthesized in the kidney by cortical interstitial cells. Although Hgb levels are normally very stable, decreased O2 delivery, low Hgb concentration, and low Pao2 stimulate the secretion of erythropoietin. This increases the production of red blood cells. Chronic renal disease damages the cortical interstitial cells and thereby suppresses their ability to synthesize erythropoietin. This causes anemia, along with decreased Hgb because of the lack of erythropoietin. Erythropoietin replacement therapy effectively increases red blood cell production.
|
Glucose Metabolism and CO2 Production
|
CO2 is produced at a rate of approximately 200 mL/min under healthy conditions, and typically, 80 molecules of CO2 will be expired by the lung for every 100 molecules of O2 that enter the capillary bed. The ratio of expired CO2 to O2 uptake is referred to as the respiratory exchange ratio and, under normal conditions, is 0.8 (80 CO2 to 100 O2). This ratio is similar at the tissue to blood compartment, where it is referred to as the respiratory quotient.
|
page 464 |  | page 465 |
The body has enhanced storage capabilities for CO2 as compared with O2, and hence Pao2 is much more
sensitive to changes in ventilation than Paco2 is. Whereas Pao2 is dependent on several factors, in addition to alveolar ventilation, arterial Paco2 is solely dependent on alveolar ventilation and CO2 production. There is an inverse relationship between alveolar ventilation and Paco2.
|
Bicarbonate and CO2 Transport
|
In blood CO2 is transported in red blood cells primarily as bicarbonate (HCO3-), but also as dissolved CO2 and as carbamino protein complexes (i.e., CO2 binds to plasma proteins and to Hgb) (Fig. 23-8). Once CO2 diffuses through the tissue and enters plasma, it quickly dissolves. The reaction of CO2 with H2O to form carbonic acid (H2CO3) provides the major pathway for the generation of HCO3- in red blood cells (Equation 23-4):
|
Figure 23-8 Mechanisms of CO2 transport in blood. The predominant mechanism by which CO2 is transported from tissue cells to the lung is in the form of HCO3-. RBC, red blood cell. |
This reaction normally proceeds quite slowly; however, it is catalyzed within red blood cells by the
enzyme carbonic anhydrase. The HCO3- diffuses out of the red blood cell in exchange for Cl-, the chloride shift, which helps the cell maintain its osmotic equilibrium.
|
The chemical reaction just described and in Figure 23-8 is reversible and can be shifted to the right to generate more HCO3- in response to more CO2 entering the blood from tissues, or it can be shifted to the left as CO2 is exhaled in the lungs, thereby reducing HCO3-. The free H+ is quickly buffered within the red blood cell by binding to Hgb. Buffering of H+ ion is critical to keep the reaction moving toward the synthesis of HCO3-; high levels of free H+ (low pH) will shift the reaction to the left.
|
REGULATION OF H+ ION CONCENTRATION AND ACID-BASE BALANCE
|
page 465 |  | page 466 |
The H+ ion concentration (pH) has a dramatic effect on many metabolic processes within cells, and regulation of pH is essential for normal homeostasis. In the
clinical setting, blood pH is measured to assess the concentration of H+. The normal pH range for adults is 7.35 to 7.45 and is maintained by the lung, kidney, and chemical buffer systems (see Chapter 36). In the respiratory system, conversion of CO2 to HCO3-, as illustrated below, provides a major mechanism to buffer and regulate the H+ concentration (pH):
|
As Paco2 changes, so does the concentration of HCO3- and H2CO3, as well as Paco2.
|
The Henderson-Hasselbalch equation is used to calculate how changes in CO2 and HCO3- affect pH.
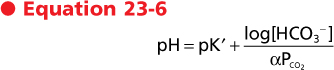 or
|
In these equations the amount of CO2 is determined from the partial pressure of CO2 (Pco2) and its solubility (α) in solution. For plasma at 37° C, α has a value of 0.03. Also, pK' is the negative logarithm of the overall dissociation constant for the reaction and has a value of 6.1 for plasma at 37° C.
|
Acute hyperventilation secondary to exercise or anxiety reduces Pco2, thereby increasing pH (respiratory alkalosis). Conversely, if Pco2 increases because of hypoventilation secondary to an overdose of a respiratory depressant, the pH decreases (respiratory acidosis). Acid-base imbalances are also caused by metabolic disorders such as metabolic acidosis (e.g., lactic acidosis, ketoacidosis, and renal failure; see Chapter 36) and metabolic alkalosis (e.g., hypokalemia, hypochloremia, vomiting, high doses of steroids; see Chapter 36).
|
THE CO2 DISSOCIATION CURVE
|
Figure 23-9 Blood CO2 equilibrium curves (arterial and venous). Venous blood can transport more CO2 than arterial blood can at any given Pco2. When compared with the HgbO2 equilibrium curve, the CO2 curves are essentially straight lines between a Pco2 of 20 and 80 mm Hg. |
In contrast to O2, the dissociation curve for CO2 in blood is linear and directly related to Pco2 (Fig. 23-9). The degree of Hgb saturation with O2 has a major effect on the CO2 dissociation curve. Although O2 and CO2 bind to Hgb at different sites, deoxygenated Hgb has greater affinity for CO2 than oxygenated Hgb does. Thus, deoxygenated blood (venous blood) freely takes up and transports more CO2 than oxygenated arterial blood does. The deoxygenated Hgb more readily forms carbamino compounds and also more readily binds free H+ ions released during the formation of HCO3-. The effect of changes in oxyhemoglobin saturation on the relationship of CO2 content to Pco2 is referred to as the Haldane effect and is reversed in the lung when O2 is transported from the alveoli to red blood cells.
This effect is illustrated by a shift to the left in the CO2 dissociation curve in venous blood as compared with arterial blood.
|
page 466 |  | page 467 |
- Diffusion and transport of O2 and CO2 are determined by basic gas diffusion laws and depend on differential pressure gradients.
- Gases (nitrous oxide, ether, helium) that have a rapid rate of air-to-blood equilibration are perfusion limited. Gases (CO) that have a slow air-to-blood equilibration rate are diffusion limited. Under normal conditions, O2 transport is perfusion limited but can be diffusion limited in some situations.
- DLCO is a classic measurement of the diffusion capabilities of the alveolar-capillary membrane. It is useful in the diagnosis of restrictive lung diseases such as interstitial pulmonary fibrosis and in distinguishing between chronic bronchitis and emphysema.
- The major transport mechanism of O2 in blood is within the red blood cell bound to Hgb, and for CO2 it is within red blood cells in the form of HCO3-.
- Tissue hypoxia occurs when insufficient amounts of O2 are supplied to the tissue to conduct normal levels of aerobic metabolism.
- The reversible reaction of CO2 with H2O to form H2CO3 with its subsequent dissociation to HCO3- and H+ is catalyzed by the enzyme carbonic anhydrase within red blood cells and is the major pathway for generation of HCO3-.
- The CO2 dissociation curve from blood is linear and directly related to Pco2. Pco2 is solely dependent on alveolar ventilation and CO2 production.
- The O2 dissociation curve is S shaped. In the plateau area (above 60 mm Hg), increasing or decreasing Po2 has only a minimal effect on Hgb saturation from 100 to 60 mm Hg. This ensures adequate Hgb saturation over a large range of Po2 values
- The CO2-to-HCO3- pathway plays a critical role in the regulation of H+ ions and in maintaining acid-base balance in the body.
|
 |
|