SECTION SEVEN THE RENAL SYSTEM
|
page 555 |  | page 556 |
page 556 |  | page 557 |
32 Elements of Renal Function
|
OVERVIEW OF RENAL FUNCTION
|
The kidney presents in the highest degree the phenomenon of sensibility, the power of reacting to various stimuli in a direction which is appropriate for the survival of the organism; a power of adaptation which almost gives one the idea that its component parts must be endowed with intelligence. E. STARLING-1909
|
Certainly, mental integrity is a sine qua non of the free and independent life. But let the composition of our internal environment suffer change, let our kidneys fail for even a short time to fulfill their tasks, and our mental integrity, or personality is destroyed. HOMER W. SMITH-1939
|
As both Starling and Smith recognized, the kidneys are regulatory rather than excretory organs. However, it is clear that the excretory function of the kidneys is central to their ability to regulate the composition and volume of body fluids. The kidneys regulate (1) body fluid osmolality and volumes, (2) electrolyte balance, and (3) acid-base balance. In addition, the kidneys excrete metabolic products and foreign substances and produce and secrete hormones.
|
Control of body fluid osmolality is important for maintenance of normal cell volume in all tissues of the body. Control of body fluid volume is necessary for normal function of the cardiovascular system. The kidneys are also essential in regulating the amount of several important inorganic ions in the body, including Na+, K+, Cl-, bicarbonate (HCO3-), hydrogen (H+), Ca++, and inorganic phosphate (Pi). Excretion of these electrolytes must be equal to daily intake of them to maintain appropriate balance. If intake of an electrolyte exceeds its excretion, the amount of this electrolyte in the body increases, and the individual is in positive balance for that electrolyte. Conversely, if excretion of an electrolyte exceeds its intake, its amount in the body decreases, and the individual is in negative balance for that electrolyte. For many electrolytes the kidneys are the sole or primary route for excretion from the body.
|
Another important function of the kidneys is regulation of acid-base balance. Many of the metabolic functions of the body are exquisitely sensitive to pH. Thus, the pH of body fluids must be maintained within narrow limits. The pH is maintained by buffers within the body fluids and by the coordinated action of the lungs, liver, and kidneys.
|
The kidneys excrete a number of the end products of metabolism. These waste products include urea (from amino acids), uric acid (from nucleic acids), creatinine (from muscle creatine), end products of hemoglobin metabolism, and metabolites of hormones. The kidneys eliminate these substances from the body at a rate that matches their production. Thus, the kidneys regulate hormone concentrations within the body fluids. The kidneys also represent an important route for the elimination of foreign substances such as drugs, pesticides, and other chemicals from the body.
|
Finally, the kidneys are important endocrine organs that produce and secrete renin, calcitriol, and erythropoietin. Renin activates the renin-angiotensin-aldosterone system, which helps regulate blood pressure and Na+ and K+ balance. Calcitriol, a metabolite of vitamin D3, is necessary for the normal absorption of Ca++ by the gastrointestinal tract and for its deposition in bone (see also Chapter 35). In patients with renal disease, the kidneys' ability to produce calcitriol is impaired, and levels of this hormone are reduced. As a result, Ca++ absorption by the intestine is decreased. This reduced intestinal Ca++ absorption contributes to the bone formation abnormalities seen in patients with chronic renal disease. Another consequence of many kidney diseases is a reduction in erythropoietin production and secretion. Erythropoietin stimulates red blood cell formation by the bone marrow. Decreased erythrocyte production contributes to the anemia that occurs in chronic renal failure.
|
A large variety of diseases impair the function of the kidneys and result in renal failure. In some instances the impairment in renal function is transient, but in many cases renal function declines progressively. Patients in whom the glomerular filtration rate (GFR) is less than 10% of normal are said to have end-stage renal disease (ESRD) and must undergo renal replacement therapy to survive.
|
To understand the mechanisms that contribute to renal disease, it is first necessary to understand the normal physiology of renal function. Thus, in the following chapters in this section of the book various aspects of renal function are considered.
|
page 557 |  | page 558 |
Kidney disease is a major health problem. In the United States:
- Kidney disease affects over 20 million patients and accounts for more than 80,000 deaths per year.
- Each year kidney disease is diagnosed in more than 3 million new patients.
- Over 500,000 people are treated for ESRD every year.
- Approximately 275,000 patients with ESRD are maintained on either hemodialysis or peritoneal dialysis.
- Diabetes, hypertension, glomerulonephritis, and polycystic kidney disease are the leading causes of ESRD.
- ESRD secondary to diabetes is increasing at an annual rate of more than 11% per year.
- The health care cost for ESRD is more than $19 billion dollars per year.
- More than 14,000 kidney transplants are performed each year. Unfortunately, in excess of 54,000 patients are awaiting kidney transplants.
- Urinary tract infections, kidney stones (i.e., urolithiasis), and interstitial cystitis (i.e., inflammation of the urinary bladder) are also major health care problems. Interstitial cystitis (700,000 patients), urinary stones (1.3 million visits annually), urinary tract infections (8.3 million visits annually), and urinary incontinence (13 million adults affected, mostly older than 65) are serious health concerns.
|
Individuals with ESRD must undergo renal replacement therapy. Such therapy includes peritoneal dialysis, hemodialysis, and renal transplantation. Both peritoneal dialysis and hemodialysis, as their names indicate, are based on the process of dialysis whereby small molecules are removed from the blood by diffusion across a selectively permeable membrane into a solution that lacks these small molecules. In peritoneal dialysis, the peritoneal membrane acts as a dialyzing membrane. Several liters of a solution are introduced into the abdominal cavity, and small molecules in blood diffuse across the peritoneal membrane into the solution, which is then removed from the abdominal cavity. In hemodialysis, a patient's blood is pumped through an artificial kidney machine. In the kidney machine blood is separated from an artificial solution by a dialysis membrane, which allows small molecules to diffuse from blood into the dialysis solution, thereby removing the small molecules from the blood. Patients who are candidates for renal transplantation are treated with dialysis until an appropriate donor kidney can be obtained. Although anemia also used to be a significant problem because of reduced erythropoietin production in ESRD, patients undergoing chronic dialysis now receive recombinant human erythropoietin. |
 |
FUNCTIONAL ANATOMY OF THE KIDNEYS
|
Structure and function are closely linked in the kidneys. Consequently, an appreciation of the gross anatomic and histological features of the kidneys is a prerequisite for understanding their function.
|
The kidneys are paired organs that lie on the posterior wall of the abdomen behind the peritoneum on either side of the vertebral column. In an adult human, each kidney weighs between 115 and 170 g and is approximately 11 cm long, 6 cm wide, and 3 cm thick.
|
The gross anatomic features of the human kidney are illustrated in Figure 32-1. The medial side of each kidney contains an indentation through which pass the renal artery and vein, nerves, and pelvis. If a kidney were cut in half, two regions would be evident: an outer region called the cortex and an inner region called the medulla. The cortex and medulla are composed of nephrons (the functional units of the kidney), blood vessels, lymphatics, and nerves. The medulla in the human kidney is divided into conical masses called renal pyramids. The base of each pyramid originates at the corticomedullary border, and the apex terminates in a papilla, which lies within a minor calyx. Minor calyces collect urine from each papilla. The numerous minor calyces expand into two or three open-ended pouches, the major calyces. The major calyces in turn feed into the pelvis. The pelvis represents the upper, expanded region of the ureter, which carries urine from the pelvis to the urinary bladder. The walls of the calyces, pelvis, and ureters contain smooth muscle that contracts to propel the urine toward the urinary bladder.
|
Blood flow to the two kidneys is equivalent to about 25% (1.25 L/min) of the cardiac output in resting individuals. However, the kidneys constitute less than 0.5% of total body weight. As illustrated in Figure 32-2 (left), the renal artery branches progressively to form the interlobar artery, the arcuate artery, the interlobular artery, and the afferent arteriole, which leads into the glomerular capillaries (i.e., glomerulus). The glomerular capillaries come together to form the efferent arteriole, which leads into a second capillary network, the peritubular capillaries, which supply blood to the nephron. The vessels of the venous system run parallel to the arterial vessels and progressively form the interlobular vein, arcuate vein, interlobar vein, and renal vein, which courses beside the ureter.
|
Ultrastructure of the Nephron
|
page 558 |  | page 559 |
Figure 32-1 Structure of a human kidney, cut open to show the internal structures. (Modified from Marsh DJ: Renal Physiology. New York, Raven, 1983.) |
|
Figure 32-2 Left, Organization of the vascular system of the human kidney. 1, interlobar arteries; 1a, interlobar vein; 2, arcuate arteries; 2a, arcuate veins; 3, interlobular arteries; 3a, interlobular veins; 4, stellate vein; 5, afferent arterioles; 6, efferent arterioles; 7a, 7b, glomerular capillary networks; 8, descending vasa recta; 9, ascending vasa recta. Right, Organization of the human nephron. A superficial nephron is illustrated on the left and a juxtamedullary (JM) nephron is illustrated on the right. The loop of Henle includes the straight portion of the proximal tubule (PT), descending thin limb (DTL), ascending thin limb (ATL), and thick ascending limb (TAL). B, Bowman's capsule; CCD, cortical collecting duct; DT, distal tubule; IMCD, inner medullary collecting duct; MD, macula densa; OMCD, outer medullary collecting duct; P, pelvis. (Modified from Kriz W, Bankir LA: Am J Physiol 254:F1, 1988; and Koushanpour E, Kriz W: Renal Physiology: Principles, Structure, and Function, 2nd ed. New York, Springer-Verlag, 1986.). |
page 559 |  | page 560 |
The functional unit of the kidneys is the nephron. Each human kidney contains approximately 1.2 million nephrons, which are hollow tubes composed of a single cell layer. The nephron consists of a renal corpuscle, proximal tubule, loop of Henle, distal tubule, and collecting duct system* (Fig. 32-3; also
see Fig. 32-4). The renal corpuscle consists of glomerular capillaries and Bowman's capsule. The proximal tubule initially forms several coils, followed by a straight piece that descends toward the medulla. The next segment is the loop of Henle, which is composed of the straight part of the proximal tubule, the descending thin limb (which ends in a hairpin turn), the ascending thin limb (only in nephrons with long loops of Henle), and the thick ascending limb. Near the end of the thick ascending limb, the nephron passes between the afferent and efferent arterioles of the same nephron. This short segment of the thick ascending limb is called the macula densa. The distal tubule begins a short distance beyond the macula densa and extends to the point in the cortex where two or more nephrons join to form a cortical collecting duct. The cortical collecting duct enters the medulla and becomes the outer medullary collecting duct and then the inner medullary collecting duct.
|
Each nephron segment is made up of cells that are uniquely suited to perform specific transport functions (Fig. 32-3). Proximal tubule cells have an extensively amplified apical membrane (the urine side of the cell) called the brush border, which is present only in the proximal tubule. The basolateral membrane (the blood side of the cell) is highly invaginated. These invaginations contain many mitochondria. In contrast, the descending and ascending thin limbs of Henle's loop have poorly developed apical and basolateral surfaces and few mitochondria. The cells of the thick ascending limb and the distal tubule have abundant mitochondria and extensive infoldings of the basolateral membrane.
|
Figure 32-3 Diagram of a nephron, including the cellular ultra-structure. |
The collecting duct is composed of two cell types: principal cells and intercalated cells. Principal cells have a moderately invaginated basolateral membrane and contain few mitochondria. Principal cells play an important role in reabsorption of NaCl (see Chapters 33 and 34) and secretion of K+ (see Chapter 35). Intercalated cells, which play an important role in regulating
acid-base balance, have a high density of mitochondria. One population of intercalated cells secretes H+ (i.e., reabsorbs HCO3-), and a second population secretes HCO3- (see Chapter 36). The final segment of the nephron, the inner medullary collecting duct, is composed of inner medullary collecting duct cells. Cells of the inner medullary collecting duct have poorly developed apical and basolateral surfaces and few mitochondria.
|
All cells in the nephron, except intercalated cells, have in their apical plasma membrane a single nonmotile primary cilium that protrudes into the tubule fluid (Fig. 32-4). Primary cilia are mechanosensors (i.e., they sense changes in the rate of flow of tubule fluid) and chemosensors (i.e., they sense or respond to compounds in the surrounding fluid), and they initiate Ca++-dependent signaling pathways, including those that control kidney cell function, proliferation, differentiation, and apoptosis (i.e., programmed cell death).
|
Polycystin 1 (encoded by the PKD1 gene) and polycystin 2 (encoded by the PKD2 gene) are expressed in the membrane of primary cilia and mediate entry of Ca++ into cells. PKD1 and PKD2 are thought to play an important role in flow-dependent K+ secretion by principal cells of the collecting duct. As described in more detail in Chapter 35, increased flow of tubule fluid in the collecting duct is a strong stimulus for secretion of K+. Increased flow bends the primary cilium in principal cells, which activates the PKD1/PKD2 Ca++ conducting channel complex and allows Ca++ to enter the cell and increase intracellular [Ca++]. The increase in [Ca++] activates K+ channels in the apical plasma membrane, which enhances secretion of K+ from the cell into the tubule fluid. |
page 560 |  | page 561 |
Polycystic kidney disease (PKD) is a genetic disease that occurs in about 1 in 800 people. Approximately 4 to 6 million people worldwide (600,000 in the United States) have PKD, which is caused primarily by mutations in PKD1 (85% to 90% of cases) and PKD2 (10% to 15% of cases). The major phenotype of PKD is enlargement of the kidneys because of the presence of hundreds to thousands of renal cysts that can be as large as 20 cm in diameter. Cysts are also seen in the liver and other organs. PKD causes renal failure, usually in the fifth decade of life, and accounts for 10% of patients with end-stage renal failure. Although it is not clear how mutations in PKD1 and PKD2 cause PKD, renal cyst formation may result from defects in uptake of Ca++ that lead to alterations in Ca++-dependent signaling pathways, including those that control kidney cell proliferation, differentiation, and apoptosis. |
 |
|
Figure 32-4 Scanning electron micrograph illustrating primary cilia (C) in the apical plasma membrane of principal cells in the cortical collecting duct. Note that intercalated cells do not have cilia. Primary cilia are approximately 2 to 30 μm long and 0.5 μm in diameter. CD, collecting duct principal cells with short microvilli (arrowhead); the straight ridges (open arrow) represent the cell borders between principal cells; IC1 and IC2, intercalated cells with numerous long microvilli in the apical membrane. (From Kriz W, Kaissling B: Structural organization of the mammalian kidney. In Seldin DW, Giebisch G [eds]: The Kidney: Physiology and Pathophysiology, 3rd ed. Philadelphia, Lippincott Williams & Wilkins, 2000.) |
Nephrons may be subdivided into superficial and juxtamedullary types (Fig. 32-2). The renal corpuscle of each superficial nephron is located in the outer region of the cortex. Its loop of Henle is short, and its efferent arteriole branches into peritubular capillaries that surround the nephron segments of its own and adjacent nephrons. This capillary network conveys oxygen and important nutrients to the nephron segments in the cortex, delivers substances to the nephron for secretion (i.e., movement of a substance from blood into tubular fluid), and serves as a pathway for return of reabsorbed water and solutes to the circulatory system. A few species, including humans, also possess very short superficial nephrons whose loops of Henle never enter the medulla.
|
The renal corpuscle of each juxtamedullary nephron is located in the region of the cortex adjacent to the medulla (Fig. 32-2, right). When compared with superficial nephrons, juxtamedullary nephrons differ anatomically in two important ways: the loop of Henle is longer and extends deeper into the medulla, and the efferent arteriole forms not only a network of peritubular capillaries but also a series of vascular loops called the vasa recta.
|
As shown in Figure 32-2, the vasa recta descend into the medulla, where they form capillary networks that surround the collecting ducts and ascending limbs of the loop of Henle. The blood returns to the cortex in the ascending vasa recta. Although less than 0.7% of the renal blood flow (RBF) enters the vasa recta, these vessels subserve important functions in the renal medulla, including (1) conveying oxygen and important nutrients to nephron segments, (2) delivering substances to the nephron for secretion, (3) serving as a pathway for the return of reabsorbed water and solutes to the circulatory system, and (4) concentrating and diluting the urine (urine concentration and dilution are discussed in more detail in Chapter 34).
|
Ultrastructure of the Renal Corpuscle
|
The first step in urine formation begins with passive movement of a plasma ultrafiltrate from the glomerular capillaries (i.e., glomerulus) into Bowman's space. The term ultrafiltration refers to the passive movement of an essentially protein-free fluid from the glomerular capillaries into Bowman's space. To appreciate the process of ultrafiltration one must understand the anatomy of the renal corpuscle. The glomerulus consists of a network of capillaries supplied by the afferent arteriole and drained by the efferent arteriole (Figs. 32-5 and 32-6). During embryological development, the glomerular capillaries press into the closed end of the proximal tubule to form the Bowman capsule of a renal corpuscle. The capillaries are covered by epithelial cells called podocytes that form the visceral layer of Bowman's capsule (Figs. 32-7 through 32-9). The visceral cells face outward at the vascular pole (i.e., where the afferent and efferent arterioles enter and exit Bowman's capsule) to form the parietal layer of Bowman's capsule. The space between the visceral layer and the parietal layer is Bowman's space, which at the urinary pole (i.e., where the proximal tubule joins Bowman's capsule) of the glomerulus becomes the lumen of the proximal tubule.
|
page 561 |  | page 562 |
Figure 32-5 Anatomy of the renal corpuscle and juxtaglomerular apparatus. The juxtaglomerular apparatus is composed of the macula densa (MD) of the thick ascending limb, extraglomerular mesangial cells (EGM), and renin- and angiotensin II-producing granular cells (G) of the afferent arterioles (AA). BM, basement membrane; BS, Bowman's space; EA, efferent arteriole; EN, endothelial cell; FP, foot processes of the podocyte; M, mesangial cells between capillaries; P, podocyte cell body (visceral cell layer); PE, parietal epithelium; PT, proximal tubule cell. (Modified from Kriz W, Kaissling B. In Seldin DW, Giebisch G [eds]: The Kidney: Physiology and Pathophysiology, 2nd ed. New York, Raven, 1992.) |
Figure 32-6 Scanning electron micrograph of the interlobular artery, afferent arteriole (af), efferent arteriole (ef), and glomerulus. The white bars on the afferent and efferent arterioles indicate that they are about 15 to 20 μm wide. (From Kimura K et al: Am J Physiol 259:F936, 1990.) |
|
Figure 32-7 A, Electron micrograph of a podocyte surrounding a glomerular capillary. The cell body of the podocyte contains a large nucleus with three indentations. Cell processes of the podocyte form the interdigitating foot processes (FP). The arrows in the cytoplasm of the podocyte indicate the well-developed Golgi apparatus, and the asterisks indicate Bowman's space. C, capillary lumen; GBM, glomerular basement membrane. B, Electron micrograph of the filtration barrier of a glomerular capillary. The filtration barrier is composed of three layers: the endothelium, basement membrane, and foot processes of the podocytes. Note the filtration slit diaphragm bridging the floor of the filtration slits (arrows). CL, capillary lumen. (From Kriz W, Kaissling B. In Seldin DW, Giebisch G [eds]: The Kidney: Physiology and Pathophysiology, 2nd ed. New York, Raven, 1992.) |
page 562 |  | page 563 |
Figure 32-8 A, Scanning electron micrograph showing the outer surface of glomerular capillaries. This is the view that would be seen from Bowman's space. Processes (P) of podocytes run from the cell body (CB) toward the capillaries, where they ultimately split into foot processes. Interdigitation of the foot processes creates the filtration slits. B, Scanning electron micrograph of the inner surface (blood side) of a glomerular capillary. This view would be seen from the lumen of the capillary. The fenestrations of the endothelial cells are seen as small 700-Å holes. (From Kriz W, Kaissling B. In Seldin DW, Giebisch G [eds]: The Kidney: Physiology and Pathophysiology, 2nd ed. New York, Raven, 1992.). |
|
Figure 32-9 Electron micrograph of the mesangium, the area between the glomerular capillaries containing mesangial cells. C, glomerular capillaries; cGBM, capillary glomerular basement membrane surrounded by foot processes of podocytes (PO) and endothelial cells; M, mesangial cell that gives rise to several processes, some marked by stars; mGBM, mesangial glomerular basement membrane surrounded by foot processes of podocytes and mesangial cells; US, urinary space. Note the extensive extracellular matrix surrounded by mesangial cells (triangles) (×4100). (From Kriz W, Kaissling B. In Seldin DW, Giebisch G [eds]: The Kidney: Physiology and Pathophysiology, 2nd ed. New York, Raven, 1992.) |
The endothelial cells of glomerular capillaries are covered by a basement membrane that is surrounded by podocytes (Figs. 32-5 and 32-7 to 32-9). The capillary endothelium, basement membrane, and foot processes of podocytes form the so-called filtration barrier (Figs. 32-5 and 32-7 to 32-9). The endothelium is fenestrated (i.e., contains 700-Å holes, where 1 Å = 10-10 m) and freely permeable to water, small solutes (such as Na+, urea, and glucose), and most proteins but is not permeable to red blood cells, white blood cells, or platelets. Because endothelial cells express
negatively charged glycoproteins on their surface, they may retard the filtration of very large anionic proteins into Bowman's space. In addition to their role as a barrier to filtration, the endothelial cells synthesize a number of vasoactive substances (e.g., nitric oxide [NO], a vasodilator, and endothelin-1 [ET-1], a vasoconstrictor) that are important in controlling renal plasma flow (RPF).
|
page 563 |  | page 564 |
|
Figure 32-10 Anatomy of podocyte foot processes. This figure illustrates the proteins that make up the slit diaphragm between two adjacent foot processes. Nephrin and NEPH1 are membrane-spanning proteins that have large extracellular domains that interact. Podocin, also a membrane-spanning protein, organizes nephrin and NEPH1 in specific microdomains in the plasma membrane, which is important for signaling events that determine the structural integrity of podocyte foot processes. Many of the proteins that compose the slit diaphragm interact with adapter proteins inside the cell, including CD2-AP. The adapter proteins bind to the filamentous actin (F-actin) cytoskeleton, which in turn binds either directly or indirectly to proteins such as α3β1 and MAGI-1 that interact with proteins expressed by the glomerular basement membrane (GBM). α-act-4, α-actinin 4; α3β1, α3β1 integrin; α-DG, α-dystroglycan; CD2-AP, an adapter protein that links nephrin and podocin to intracellular proteins; FAT, a protocadherin that organizes actin polymerization; MAGI-1, a membrane-associated guanylate kinase protein; NHERF-2, Na+-H+ exchanger regulatory factor 2; P, paxillin; P-Cad, P-cadherin; Synpo, synaptopodin; T, talin; V, vinculin; Z, zona occludens. (Adapted from Mundel P, Shankland SJ: J Am Soc Nephrol 13:3005, 2002.) |
The basement membrane, which is a porous matrix of negatively charged proteins, including type IV collagen, laminin, the proteoglycans agrin and perlecan, and fibronectin, is an important filtration barrier to plasma proteins. The basement membrane is thought to function primarily as a charge-selective filter in
which the ability of proteins to cross the filter is based on charge.*
|
The podocytes, which are endocytic, have long finger-like processes that completely encircle the outer surface of the capillaries (Fig. 32-8). The processes of the podocytes interdigitate to cover the basement membrane and are separated by apparent gaps called filtration slits. Each filtration slit is bridged by a thin diaphragm that contains pores with a dimension of 40 × 140 Å. The filtration slit diaphragm, which appears as a continuous structure when viewed by electron microscopy (Fig. 32-7, B), is composed of several proteins, including nephrin (NPHS1), NEPH-1, podocin (NPHS2), α-actinin 4 (ACTN4), and CD2-AP (Figs. 32-10 and 32-11). Filtration slits, which function primarily as a size-selective filter, keep the proteins and macromolecules that cross the basement membrane from entering Bowman's space.
|
Nephrotic syndrome is produced by a variety of disorders and is characterized by an increase in permeability of the glomerular capillaries to proteins and by loss of normal podocyte structure, including effacement (i.e., thinning) of the foot processes. The augmented permeability to proteins results in an increase in urinary protein excretion (proteinuria). Thus, the appearance of proteins in urine can indicate kidney disease. Hypoalbuminemia often develops in individuals with this syndrome as a result of the proteinuria. In addition, generalized edema is commonly seen in individuals with nephrotic syndrome. Mutations in several genes that encode slit diaphragm proteins (Figs. 32-10 and 32-11), including nephrin, NEPH-1, podocin, CD2-AP, and α-actinin 4, or knockout of these genes in mice causes proteinuria and kidney disease. For example, mutations in the nephrin gene (NPHS1) lead to abnormal or absent slit diaphragms, which causes massive proteinuria and renal failure (i.e., congenital nephrotic syndrome). In addition, mutations in the podocin gene (NPHS2) cause autosomal recessive, steroid-resistant nephrotic syndrome. These naturally occurring mutations and knockout studies in mice demonstrate that nephrin, NEPH-1, podocin, CD2-AP, and α-actinin 4 play a key role in podocyte structure and function. |
 |
page 564 |  | page 565 |
Figure 32-11 Overview of the major proteins that form the slit diaphragm. Nephrons (red) from opposite foot processes interdigitate in the center of the slit. In the slit, nephrin interacts with NEPH1 and NEPH2 (blue), FAT1 and FAT2 (green), and P-cadherin. The intracellular domains of nephrin, NEPH1, and NEPH2 interact with podocin and CD2-AP, which connect these slit diaphragm proteins with ZO-1, α-actinin 4, and actin. (Modified from Tryggvason K et al: N Engl J Med 354:1387, 2006.) |
Alport's syndrome is characterized by hematuria (i.e., blood in urine) and progressive glomerulonephritis (i.e., inflammation of the glomerular capillaries) and accounts for 1% to 2% of all cases of ESRD. Alport's syndrome is caused by defects in type IV collagen (encoded by the COL4A5 gene), a major component of the glomerular basement membrane. In about 85% of patients with Alport's syndrome, the disease is X-linked with mutations in the COL4A5 gene. The remaining 15% of patients also have mutations in type IV collagen genes; six have been identified, but the mode of inheritance is autosomal recessive. In Alport's syndrome the glomerular basement membrane becomes irregular in thickness and fails to serve as an effective filtration barrier to blood cells and protein. |
 |
Another important component of the renal corpuscle is the mesangium, which consists of mesangial cells and the mesangial matrix (Fig. 32-9). Mesangial cells, which possess many properties of smooth muscle cells, surround the glomerular capillaries, provide structural support for the glomerular capillaries,
secrete the extracellular matrix, exhibit phagocytic activity by removing macromolecules from the mesangium, and secrete prostaglandins and proinflammatory cytokines. Because they also contract and are adjacent to glomerular capillaries, mesangial cells may influence the GFR by regulating blood flow through the glomerular capillaries or by altering the capillary surface area. Mesangial cells located outside the glomerulus (between the afferent and efferent arterioles) are called extraglomerular mesangial cells.
|
Ultrastructure of the Juxtaglomerular Apparatus
|
The juxtaglomerular apparatus is one component of an important feedback mechanism described later in the chapter, the tubuloglomerular feedback mechanism. Structures that make up the juxtaglomerular apparatus include the following (Fig. 32-5):
|
page 565 |  | page 566 |
Mesangial cells are involved in the development of immune complex-mediated glomerular disease. Because the glomerular basement membrane does not completely surround all glomerular capillaries (Fig. 32-9), some immune complexes can enter the mesangial area without crossing the glomerular basement membrane. Accumulation of immune complexes induces the infiltration of inflammatory cells into the mesangium and promotes the production of proinflammatory cytokines and autocoids by cells in the mesangium. These cytokines and autocoids enhance the inflammatory response. This inflammatory response can lead to cell scarring and eventually obliterates the glomerulus. |
- The macula densa of the thick ascending limb
- Extraglomerular mesangial cells
- Renin- and angiotensin II-producing granular cells of the afferent arteriole
|
The cells of the macula densa represent a morphologically distinct region of the thick ascending limb. This region passes through the angle formed by the afferent and efferent arterioles of the same nephron. The cells of the macula densa contact the extraglomerular mesangial cells and the granular cells of the afferent arterioles. The granular cells of the afferent arterioles are derived from metanephric mesenchymal cells. They contain smooth muscle myofilaments, and importantly, they manufacture, store, and release renin. Renin is involved in the formation of angiotensin II and ultimately in the secretion of aldosterone (see Chapter 34). The juxtaglomerular apparatus is one component of the tubuloglomerular feedback mechanism that is involved in the autoregulation of RBF and GFR.
|
Innervation of the Kidneys
|
Renal nerves regulate RBF, GFR, and salt and water reabsorption by the nephron. The nerve supply to the kidneys consists of sympathetic nerve fibers that originate in the celiac plexus. There is no parasympathetic innervation. Adrenergic fibers that innervate the kidneys release norepinephrine and dopamine. The adrenergic fibers lie adjacent to the smooth muscle cells of the major branches of the renal artery (interlobar, arcuate, and interlobular arteries) and the afferent and efferent arterioles. Moreover, sympathetic nerves innervate the renin-producing granular cells of the afferent arterioles. Renin secretion is stimulated by increased sympathetic activity. Nerve fibers also innervate the proximal tubule, loop of Henle, distal tubule, and collecting duct; activation of these nerves enhances Na+ reabsorption by these nephron segments.
|
ASSESSMENT OF RENAL FUNCTION
|
Figure 32-12 Mass balance relationships for the kidney. See text for definition of symbols. |
The coordinated actions of the nephron's various segments determine the amount of a substance that appears in urine. This represents three general processes:
(1) glomerular filtration, (2) reabsorption of the substance from tubular fluid back into blood, and (3) (in some cases) secretion of the substance from blood into tubule fluid. The first step in the formation of urine by the kidneys is the production of an ultrafiltrate of plasma across the glomerulus. The process of glomerular filtration and regulation of GFR and RBF are discussed later in this chapter. The concept of renal clearance, which is the theoretical basis for measurement of GFR and RBF, is presented in the following section. Reabsorption and secretion are discussed in subsequent chapters.
|
The concept of renal clearance is based on the Fick principle (i.e., mass balance or conservation of mass). Figure 32-12 illustrates the various factors required to describe the mass balance relationships of a kidney. The renal artery is the single input source to the kidney, whereas the renal vein and ureter constitute the two output routes. The following equation defines the mass balance relationship:
 where
and
are the concentrations of substance x in the renal artery and renal vein plasma, respectively- RPFa and RPFv are renal plasma flow rates in the artery and vein, respectively
- Ux is the concentration of substance x in urine
- V is the urine flow rate
|
page 566 |  | page 567 |
This relationship permits quantification of the amount of substance x excreted in urine versus the amount returned to the systemic circulation in renal venous blood. Thus, for any substance that is neither synthesized nor metabolized, the amount that enters the kidneys is equal to the amount that leaves the
kidneys in urine plus the amount that leaves the kidneys in renal venous blood.
|
The principle of renal clearance emphasizes the excretory function of the kidneys; it considers only the rate at which a substance is excreted into urine and not its rate of return to the systemic circulation in the renal vein. Therefore, in terms of mass balance (Equation 32-1), the urinary excretion rate of substance x (Ux × V.) is proportional to the plasma concentration of substance x (
):
|
To equate the urinary excretion rate of substance x to its renal arterial plasma concentration, it is necessary to determine the rate at which it is removed from plasma by the kidneys. This removal rate is the clearance (Cx).
|
If Equation 32-3 is rearranged and the concentration of substance x in renal artery plasma (
) is assumed to be identical to its concentration in a plasma sample from any peripheral blood vessel, the following relationship is obtained:
|
Clearance has the dimensions of volume/time, and it represents a volume of plasma from which all the substance has been removed and excreted into urine per unit time. This last point is best illustrated by considering the following example. If a substance is present in urine at a concentration of 100 mg/mL and the urine flow rate is 1 mL/min, the excretion rate for this substance is calculated as follows:
|
If this substance is present in plasma at a concentration of 1 mg/mL, its clearance according to Equation 32-4 is as follows:
|
In other words, 100 mL of plasma will be completely cleared of substance x each minute. The definition of clearance as a volume of plasma from which all the substance has been removed and excreted into urine per unit time is somewhat misleading because it is not a real volume of plasma; rather, it is an idealized volume.* The concept of clearance is important because it can be used to measure GFR and RPF and determine whether a substance is reabsorbed or secreted along the nephron.
|
|
Figure 32-13 Renal handling of creatinine. Creatinine is freely filtered across the glomerulus and is, to a first approximation, not reabsorbed, secreted, or metabolized by the nephron. Note that all the creatinine coming to the kidney in the renal artery does not get filtered at the glomerulus (normally, 15% to 20% of plasma creatinine is filtered). The portion that is not filtered is returned to the systemic circulation in the renal vein. PCr, plasma creatinine concentration; RPF, renal plasma flow; Ucr, urinary concentration of creatinine; V, urine flow rate. |
Glomerular Filtration Rate
|
The GFR is equal to the sum of the filtration rates of all functioning nephrons. Thus, it is an index of kidney function. A fall in GFR generally means that the kidney disease is progressing, whereas recovery generally suggests recuperation. Thus, knowledge of the patient's GFR is essential in evaluating the severity and course of kidney disease.
|
Creatinine is a byproduct of skeletal muscle creatine metabolism, and it can be used to measure GFR.* Creatinine is freely filtered across the glomerulus into Bowman's space, and to a first approximation, it is not reabsorbed, secreted, or metabolized by the cells of the nephron. Accordingly, the amount of creatinine excreted in urine per minute equals the amount of creatinine filtered at the glomerulus each minute (Fig. 32-13):
 where
- PCr
- plasma concentration of creatinine
- UCr
- urine concentration of creatinine
- V
- urine flow
|
page 567 |  | page 568 |
Creatinine is used to estimate the GFR in clinical practice. It is synthesized at a relatively constant rate, and the amount produced is proportional to the muscle mass. However, creatinine is not a perfect substance for measuring GFR because it is secreted to a small extent by the organic cation secretory system in the proximal tubule (see Chapter 33). The error introduced by this secretory component is approximately 10%. Thus, the amount of creatinine excreted in urine exceeds the amount expected from filtration alone by 10%. However, the method used to measure the plasma creatinine concentration (PCr) overestimates the true value by 10%. Consequently, the two errors cancel, and in most clinical situations, creatinine clearance provides a reasonably accurate measure of GFR. |
If Equation 32-7 is solved for GFR,
|
This equation is the same form as that for clearance (Equation 32-4). Thus, clearance of creatinine provides a means for determining the GFR. Clearance has the dimensions of volume/time, and it represents a volume of plasma from which all the substance has been removed and excreted into urine per unit time.
|
Creatinine is not the only substance that can be used to measure GFR. Any substance that meets the following criteria can serve as an appropriate marker for the measurement of GFR. The substance must
- Be freely filtered across the glomerulus into Bowman's space
- Not be reabsorbed or secreted by the nephron
- Not be metabolized or produced by the kidney
- Not alter the GFR
|
Not all of the creatinine (or other substances used to measure GFR) that enters the kidney in renal arterial plasma is filtered at the glomerulus. Likewise, not all of the plasma coming into the kidneys is filtered. Although nearly all of the plasma that enters the kidneys in the renal artery passes through the glomerulus, approximately 10% does not. The portion of filtered plasma is termed the filtration fraction and is determined as
|
Under normal conditions the filtration fraction averages 0.15 to 0.20, which means that only 15% to 20% of the plasma that enters the glomerulus is actually filtered. The remaining 80% to 85% continues on through the glomerular capillaries and into the efferent arterioles and peritubular capillaries. It is finally returned to the systemic circulation in the renal vein.
|
A fall in GFR may be the first and only clinical sign of kidney disease. Thus, measuring GFR is important when kidney disease is suspected. A 50% loss of functioning nephrons reduces the GFR only by about 25%. The decline in GFR is not 50% because the remaining nephrons compensate. Because measurements of GFR are cumbersome, kidney function is usually assessed in the clinical setting by measuring PCr, which is inversely related to GFR (Fig. 32-14). However, as Figure 32-14 shows, GFR must decline substantially before an increase in PCr can be detected in a clinical setting. For example, a fall in GFR from 120 to 100 mL/min is accompanied by an increase in PCr from 1.0 to 1.2 mg/dL. This does not appear to be a significant change in PCr, but the GFR has actually fallen by almost 20%. |
 |
|
Figure 32-14 Relationship between GFR and plasma [creatinine] (Pcr). The amount of creatinine filtered is equal to the amount excreted; thus, GFR × PCr = UCr × V. Because the production of creatinine is constant, excretion must be constant to maintain creatinine balance. Therefore, if the GFR falls from 120 to 60 mL/min, PCr must increase from 1 to 2 mg/dL to keep the filtration of creatinine and its excretion equal to the production rate. |
page 568 |  | page 569 |
Figure 32-15 Influence of the size and electrical charge of dextran on its filterability. A value of 1 indicates that it is filtered freely, whereas a value of zero indicates that it is not filtered. The filterability of dextrans between approximately 20 and 42 Å depends on charge. Dextrans larger than 42 Å are not filtered regardless of charge, and polycationic dextrans and neutral dextrans smaller than 20 Å are freely filtered. The major proteins in plasma are albumin and immunoglobulins. Because the effective molecular radii of IgG (53 Å) and IgM (>100 Å) are greater than 42 Å, they are not filtered. Although the effective molecular radius of albumin is 35 Å, it is a polyanionic protein, so it does not cross the filtration barrier to a significant degree. |
The first step in the formation of urine is ultrafiltration of plasma by the glomerulus. In normal adults, the GFR ranges from 90 to 140 mL/min in males and from 80 to 125 mL/min in females. Thus, in 24 hours as much as 180 L of plasma is filtered by the glomeruli. The plasma ultrafiltrate is devoid of cellular elements (i.e., red and white blood cells and platelets) and is essentially protein free. The concentration of salts and organic molecules, such as glucose and amino acids, is similar
in plasma and the ultrafiltrate. Starling forces drive ultrafiltration across the glomerular capillaries, and changes in these forces alter the GFR. GFR and RPF are normally held within very narrow ranges by a phenomenon called autoregulation. The next sections of this chapter review the composition of the glomerular filtrate, the dynamics of its formation, and the relationship between RPF and GFR. In addition, factors that contribute to autoregulation and regulation of GFR and RBF are discussed.
|
Determinants of Ultrafiltrate Composition
|
The glomerular filtration barrier determines the composition of the plasma ultrafiltrate. It restricts the filtration of molecules on the basis of both size and electrical charge (Fig. 32-15). In general, neutral molecules with a radius smaller than 20 Å are filtered freely, molecules larger than 42 Å are not filtered, and molecules between 20 and 42 Å are filtered to various degrees. For example, serum albumin, an anionic protein that has an effective molecular radius of 35.5 Å, is filtered poorly. Because the filtered albumin is normally reabsorbed avidly by the proximal tubule, almost no albumin appears in urine.
|
The importance of the negative charges on the filtration barrier in restricting the filtration of plasma proteins is shown in Figure 32-16. Removal of the negative charges from the filtration barrier causes proteins to be filtered solely on the basis of their effective molecular radius. Hence, at any molecular radius between approximately 20 and 42 Å, filtration of polyanionic proteins will exceed the filtration that prevails in the normal state (in which the filtration barrier has anionic charges). In a number of glomerular diseases the negative charges on the filtration barrier are reduced because of immunological damage and inflammation. As a result, the filtration of proteins is increased, and proteins appear in urine (proteinuria). |
 |
|
Figure 32-16 Reduction of the negative charges on the glomerular wall results in the filtration of proteins on the basis of size only. In this situation the relative filterability of proteins depends only on the molecular radius. Accordingly, excretion of polyanionic proteins (20 to 42 Å) in urine increases because more proteins of this size are filtered. |
Figure 32-15 shows how electrical charge affects the filtration of macromolecules (e.g., dextrans) by the glomerulus. Dextrans are a family of exogenous polysaccharides manufactured in various molecular weights. They can be electrically neutral or have either negative charges (polyanionic) or positive charges (polycationic). As the size (i.e., effective molecular
radius) of a dextran molecule increases, the rate at which it is filtered decreases. For any given molecular radius, cationic molecules are more readily filtered than anionic molecules. The reduced filtration rate for anionic molecules is explained by the presence of negatively charged glycoproteins on the surface of all components of the glomerular filtration barrier. These charged glycoproteins repel similarly charged molecules. Because most plasma proteins are negatively charged, the negative charge on the filtration barrier restricts the filtration of proteins that have a molecular radius of 20 to 42 Å or greater.
|
Dynamics of Ultrafiltration
|
page 569 |  | page 570 |
The forces responsible for the glomerular filtration of plasma are the same as those in all capillary beds. Ultrafiltration occurs because the Starling forces (i.e.,
hydrostatic and oncotic pressure) drive fluid from the lumen of glomerular capillaries, across the filtration barrier, and into Bowman's space (Fig. 32-17). The hydrostatic pressure in the glomerular capillary (PGC) is oriented to promote the movement of fluid from the glomerular capillary into Bowman's space. Because the reflection coefficient (σ) for proteins across the glomerular capillary is essentially 1, the glomerular ultrafiltrate is protein free, and the oncotic pressure in Bowman's space (πBS) is near zero. Therefore, PGC is the only force that favors filtration. The hydrostatic pressure in Bowman's space (PBS) and the oncotic pressure in the glomerular capillary (πGC) oppose filtration.
|
As shown in Figure 32-17, a net ultrafiltration pressure (PUF) of 17 mm Hg exists at the afferent end of the glomerulus, whereas at the efferent end it is 8 mm Hg (where PUF = PGC - PBS - πGC). Two additional points concerning Starling forces and this pressure change are important. First, PGC decreases slightly along the length of the capillary because of the resistance to flow along the length of the capillary. Second, πGC increases along the length of the glomerular capillary. Because water is filtered and protein is retained in the glomerular capillary, the protein concentration in the capillary rises, and πGC increases.
|
|
Figure 32-17 Idealized glomerular capillary and the Starling forces across it. The reflection coefficient (σ) for protein across the glomerular capillary is 1. PBS, hydrostatic pressure in Bowman's space; PGC, hydrostatic pressure in the glomerular capillary; PUF, net ultrafiltration pressure; πBS, oncotic pressure in Bowman's space; πGC, oncotic pressure in the glomerular capillary. The negative signs for PBS and πGC indicate that these forces oppose formation of the glomerular filtrate. |
GFR is proportional to the sum of the Starling forces that exist across the capillaries [(PGC - PBS) - σ(πGC - πBS)] multiplied by the ultrafiltration coefficient (Kf). That is,
|
Kf is the product of the intrinsic permeability of the glomerular capillary and the glomerular surface area available for filtration. The rate of glomerular filtration is considerably greater in glomerular capillaries than in systemic capillaries, mainly because Kf is approximately 100 times greater in glomerular capillaries. Furthermore, PGC is approximately twice as great as the hydrostatic pressure in systemic capillaries.
|
GFR can be altered by changing Kf or by changing any of the Starling forces. In normal individuals, the GFR is regulated by alterations in PGC that are mediated mainly by changes in afferent or efferent arteriolar resistance. PGC is affected in three ways:
- Changes in afferent arteriolar resistance: A decrease in resistance increases PGC and GFR, whereas an increase in resistance decreases them.
- Changes in efferent arteriolar resistance: A decrease in resistance reduces PGC and GFR, whereas an increase in resistance elevates them.
- Changes in renal arteriolar pressure: An increase in blood pressure transiently increases PGC (which enhances GFR), whereas a decrease in blood pressure transiently decreases PGC (which reduces GFR).
|
A reduction in the GFR in disease states is most often due to decreases in Kf because of the loss of filtration surface area. The GFR also changes in pathophysiological conditions because of changes in PGC, πGC, and PBS.
- Changes in Kf: Increased Kf enhances the GFR, whereas decreased Kf reduces the GFR. Some kidney diseases reduce Kf by decreasing the number of filtering glomeruli (i.e., diminished surface area). Some drugs and hormones that dilate the glomerular arterioles also increase Kf. Similarly, drugs and hormones that constrict the glomerular arterioles also decrease Kf.
- Changes in PGC: With decreased renal perfusion, the GFR declines because PGC falls. As previously discussed, a reduction in PGC is caused by a decline in renal arterial pressure, an increase in afferent arteriolar resistance, or a decrease in efferent arteriolar resistance.
- Changes in πGC: An inverse relationship exists between πGC and GFR. Alterations in πGC result from changes in protein synthesis outside the kidneys. In addition, the protein loss in urine caused by some renal diseases can lead to a decrease in the plasma protein concentration and thus in πGC.
- Changes in PBS: Increased PBS reduces the GFR, whereas decreased PBS enhances the GFR. Acute obstruction of the urinary tract (e.g., a kidney stone occluding the ureter) increases PBS.
|
 |
page 570 |  | page 571 |
Blood flow through the kidneys serves several important functions, including the following:
- Indirectly determines the GFR
- Modifies the rate of solute and water reabsorption by the proximal tubule
- Participates in the concentration and dilution of urine
- Delivers O2, nutrients, and hormones to the cells of the nephron and returns CO2 and reabsorbed fluid and solutes to the general circulation
- Delivers substrates for excretion in urine
|
Blood flow through any organ may be represented by the following equation:
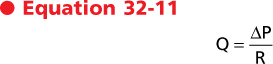 where
- Q = blood flow
- ΔP = mean arterial pressure minus venous pressure for that organ
- R = resistance to flow through that organ
|
Accordingly, RBF is equal to the pressure difference between the renal artery and the renal vein divided by renal vascular resistance:
|
The afferent arteriole, efferent arteriole, and interlobular artery are the major resistance vessels in the kidneys and thereby determine renal vascular resistance. Like most other organs, the kidneys regulate their blood flow by adjusting vascular resistance in response to changes in arterial pressure. As shown in Figure 32-18, these adjustments are so precise that blood flow remains relatively constant as arterial blood pressure changes between 90 and 180 mm Hg. GFR is also regulated over the same range of arterial pressures. The phenomenon whereby RBF and GFR are maintained relatively constant, namely, autoregulation, is achieved by changes in vascular resistance, mainly through the afferent arterioles of the kidneys. Because both GFR and RBF are regulated over the same range of pressures and because RBF is an important determinant of GFR, it is not surprising that the same mechanisms regulate both flows.
|
|
Figure 32-18 Relationship between arterial blood pressure and RBF and between arterial blood pressure and GFR. Autoregulation maintains GFR and RBF relatively constant as blood pressure changes from 90 to 180 mm Hg. |
Figure 32-19 Tubuloglomerular feedback. An increase in GFR (1) increases [NaCl] in tubule fluid in the loop of Henle (2). The increase in [NaCl] is sensed by the macula densa and converted to a signal (3) that increases the resistance of the afferent arteriole (RA) (4), which decreases the GFR. (Modified from Cogan MG: Fluid and Electrolytes: Physiology and Pathophysiology. Norwalk, CT, Appleton & Lange, 1991.) |
Two mechanisms are responsible for the autoregulation of RBF and GFR: one mechanism that responds to changes in arterial pressure and another that responds to changes in [NaCl] in tubular fluid. Both regulate the tone of the afferent arteriole. The pressure-sensitive mechanism, the so-called myogenic mechanism, is related to an intrinsic property of vascular smooth muscle: the tendency to contract when stretched. Accordingly, when arterial pressure rises and the renal afferent arteriole is stretched, the smooth muscle contracts. Because the increase in resistance
of the arteriole offsets the increase in pressure, RBF and therefore GFR remain constant. (That is, RBF is constant if ΔP/R is kept constant [Equation 32-11].)
|
page 571 |  | page 572 |
The second mechanism responsible for the autoregulation of GFR and RBF is the [NaCl]-dependent mechanism known as tubuloglomerular feedback (Fig. 32-19). This mechanism involves a feedback loop
in which the concentration of NaCl in tubular fluid is sensed by the macula densa of the juxtaglomerular apparatus (Fig. 32-20; also see Fig. 32-5) and converted into a signal or signals that affect afferent arteriolar resistance and thus the GFR. When the GFR increases and causes [NaCl] in tubular fluid at the macula densa to rise, more NaCl enters the macula densa cells. This leads to an increase in the formation and release of ATP and adenosine, a metabolite of ATP, by macula densa cells, which causes vasoconstriction of the afferent arteriole. Vasoconstriction of the afferent arteriole returns the GFR to normal levels. In contrast, when the GFR and [NaCl] in tubule fluid decrease, less NaCl enters the macula densa cells, and ATP and adenosine production and release decline. The fall in [ATP] and [adenosine] causes vasodilation of the afferent arteriole, which returns the GFR to normal. NO, a vasodilator produced by the macula densa, attenuates tubuloglomerular feedback, whereas angiotensin II enhances tubuloglomerular feedback. Thus, the macula densa may release both vasoconstrictors (e.g., ATP and adenosine) and a vasodilator (e.g., NO) that oppose each other's action at the level of the afferent arteriole. Production plus release of vasoconstrictors and vasodilators ensures exquisite control over tubuloglomerular feedback.
|
Figure 32-20 also illustrates the role of the macula densa in controlling the secretion of renin by granular cells of the afferent arteriole. This aspect of function of the juxtaglomerular apparatus is considered in detail in Chapter 34.
|
|
Figure 32-20 Cellular mechanism whereby an increase in the delivery of NaCl to the macula densa causes vasoconstriction of the afferent arteriole of the same nephron (i.e., tubuloglomerular feedback). An increase in GFR elevates [NaCl] in tubule fluid at the macula densa. This in turn enhances uptake of NaCl across the apical cell membrane of macula densa cells via the 1Na+-1K+-2Cl- (NKCC2) symporter, which leads to an increase in [ATP] and [adenosine] (ADO). ATP binds to P2X receptors and adenosine binds to adenosine A1 receptors in the plasma membrane of smooth muscle cells surrounding the afferent arteriole, both of which increase intracellular [Ca++]. The rise in [Ca++] induces vasoconstriction of the afferent arteriole, thereby returning GFR to normal levels. Note that ATP and adenosine also inhibit renin release by granular cells in the afferent arteriole. This too results from an increase in intracellular [Ca++] as a reflection of electrical coupling of the granular and vascular smooth muscle (VSM) cells. When GFR is reduced, [NaCl] in tubule fluid falls, as does uptake of NaCl into macula densa cells. This in turn decreases release of ATP and adenosine, which decreases intracellular [Ca++] and thereby increases GFR and stimulates the release of renin by granular cells. In addition, a decrease in entry of NaCl into macula densa cells enhances the production of PGE2, which also stimulates renin secretion by granular cells. As discussed in detail in Chapters 4 and 6, renin increases plasma [angiotensin II], a hormone that enhances NaCl and water retention by the kidneys. (Modified from Persson AEG et al: Acta Physiol Scand 181:471, 2004.) |
page 572 |  | page 573 |
Because animals engage in many activities that can change arterial blood pressure, mechanisms that maintain RBF and GFR relatively constant despite changes in arterial pressure are highly desirable. If the GFR and RBF were to rise or fall suddenly in proportion to changes in blood pressure, urinary excretion of fluid and solute would also change suddenly. Such changes in excretion of water and solutes without comparable changes in intake would alter the fluid and
electrolyte balance (the reason for which is discussed in Chapter 34). Accordingly, autoregulation of GFR and RBF provides an effective means for uncoupling renal function from arterial pressure, and it ensures that fluid and solute excretion remain constant.
|
Three points concerning autoregulation should be noted:
- Autoregulation is absent when arterial pressure is less than 90 mm Hg.
- Autoregulation is not perfect; RBF and GFR do change slightly as arterial blood pressure varies.
- Despite autoregulation, RBF and GFR can be changed by certain hormones and by changes in sympathetic nerve activity (Table 32-1).
|
REGULATION OF RENAL BLOOD FLOW AND THE GLOMERULAR FILTRATION RATE
|
Tubuloglomerular feedback (TGF) is absent in mice that do not express the adenosine receptor (A1). This underscores the importance of adenosine signaling in TGF. Studies have shown that when the GFR increases and causes the concentration of NaCl in tubular fluid at the macula densa to rise, more NaCl enters cells via the 1Na+-1K+-2Cl- symporter (NKCC2) located in the apical plasma membrane (Fig. 32-20). Increased intracellular [NaCl] in turn stimulates the release of ATP via ATP-conducting ion channels located in the basolateral membrane of macula densa cells. In addition, adenosine production is also enhanced. Adenosine binds to A1 receptors and ATP binds to P2X receptors located on the plasma membrane of smooth muscle cells in the afferent arteriole. Both hormones increase intracellular [Ca++], which causes vasoconstriction of the afferent artery and therefore a fall in GFR. Although adenosine is a vasodilator in most other vascular beds, it constricts the afferent arteriole in the kidney. |
 |
Table 32-1.
Major Hormones That Influence the Glomerular Filtration Rate and Renal Blood Flow |
| Stimulus | Effect on GFR | Effect on RBF |
Vasoconstrictors |
Sympathetic nerves | ↓ ECFV | ↓ | ↓ |
Angiotensin II | ↓ ECFV | ↓ | ↓ |
Endothelin | ↑ Stretch, A-II, bradykinin, epinephrine; ↓ ECFV | ↓ | ↓ |
Vasodilators |
Prostaglandins (PGE1, PGE2, PGI2) | ↓ ECFV; ↑ shear stress, A-II | No change/↑ | ↑ |
Nitric oxide (NO) | ↑ Shear stress, acetylcholine, histamine, bradykinin, ATP | ↑ | ↑ |
Bradykinin | ↑ Prostaglandins, ↓ ACE | ↑ | ↑ |
Natriuretic peptides (ANP, BNP) | ↑ ECFV | ↑ | No change |
|
A-II, angiotensin II; ECFV, extracellular fluid volume.
|
Several factors and hormones affect the GFR and RBF (Table 32-1). As discussed, the myogenic mechanism
and tubuloglomerular feedback play key roles in maintaining GFR and RBF constant. In addition, sympathetic nerves, angiotensin II, prostaglandins, NO, endothelin, bradykinin, ATP, and adenosine exert major control over RBF and GFR. Figure 32-21 shows how changes in afferent and afferent arteriolar resistance, mediated by changes in the hormones listed in Table 32-1, modulate GFR and RBF.
|
The afferent and efferent arterioles are innervated by sympathetic neurons; however, sympathetic tone is minimal when the volume of extracellular fluid is normal (see Chapter 34). Sympathetic nerves release norepinephrine and dopamine, and circulating epinephrine (a catecholamine like norepinephrine and dopamine) is secreted by the adrenal medulla. Norepinephrine and epinephrine cause vasoconstriction by binding to α1-adrenoceptors, which are located mainly on the afferent arterioles. Activation of α1-adrenoceptors decreases GFR and RBF. Dehydration or strong emotional stimuli, such as fear and pain, activate sympathetic nerves and reduce GFR and RBF.
|
Renalase, a catecholamine-metabolizing hormone produced by the kidneys, facilitates the degradation of catecholamines.
|
Individuals with renal artery stenosis (narrowing of the lumen of the artery) caused by atherosclerosis, for example, can have elevated systemic arterial blood pressure mediated by stimulation of the renin-angiotensin system (see Chapter 34). Pressure in the renal artery proximal to the stenosis is increased, but pressure distal to the stenosis is normal or reduced. Autoregulation is important in maintaining RBF, PGC, and GFR in the presence of this stenosis. Administration of drugs to lower systemic blood pressure also lowers the pressure distal to the stenosis; accordingly, RBF, PGC, and GFR fall. |
page 573 |  | page 574 |
|
Figure 32-21 Relationship between selective changes in the resistance of either the afferent arteriole or the efferent arteriole on RBF and GFR. Constriction of either the afferent or efferent arteriole increases resistance, and according to Equation 32-11 (Q = ΔP/R), an increase in resistance (R) decreases flow (Q) (i.e., RBF). Dilation of either the afferent or afferent arteriole increases flow (i.e., RBF). Constriction of the afferent arteriole (A) decreases PGC because less of the arterial pressure is transmitted to the glomerulus, thereby reducing GFR. In contrast, constriction of the efferent arteriole (B) elevates PGC and thus increases GFR. Dilation of the efferent arteriole (C) decreases PGC and thus decreases GFR. Dilation of the afferent arteriole (D) increases PGC because more of the arterial pressure is transmitted to the glomerulus, thereby increasing GFR. (Modified from Rose BD, Rennke KG: Renal Pathophysiology: The Essentials. Baltimore, Williams & Wilkins, 1994.) |
Angiotensin II is produced systemically and locally within the kidneys. It constricts the afferent and efferent
arterioles* and decreases RBF and GFR. Figure 32-22 shows how norepinephrine, epinephrine, and angiotensin II act together to decrease RBF and GFR and thereby increase blood pressure and extracellular fluid volume, as would occur, for example, with hemorrhage.
|
Hemorrhage decreases arterial blood pressure and therefore activates the sympathetic nerves to the kidneys via the baroreceptor reflex (Fig. 32-22). Norepinephrine causes intense vasoconstriction of the afferent and efferent arterioles and thereby decreases GFR and RBF. The rise in sympathetic activity also increases the release of epinephrine and angiotensin II, which cause further vasoconstriction and a fall in RBF. The rise in the vascular resistance of the kidneys and other vascular beds increases total peripheral resistance. The resulting tendency for blood pressure to increase (blood pressure = cardiac output × total peripheral resistance) offsets the tendency of blood pressure to decrease in response to hemorrhage. Hence, this system works to preserve arterial pressure at the expense of maintaining normal GFR and RBF. |
 |
Prostaglandins do not play a major role in regulating RBF in healthy, resting people. However, during pathophysiological conditions such as hemorrhage, prostaglandins (PGI2, PGE1, and PGE2) are produced locally within the kidneys, and they increase RBF without changing GFR. Prostaglandins increase RBF by dampening the vasoconstrictor effects of sympathetic nerves and angiotensin II. This effect is important because it prevents severe and potentially harmful vasoconstriction and renal ischemia. Synthesis of prostaglandins is stimulated by dehydration and stress (e.g., surgery, anesthesia), angiotensin II, and sympathetic nerves. Nonsteroidal antiinflammatory drugs (NSAIDs), such as aspirin and ibuprofen, inhibit the synthesis of prostaglandins. Thus, administration of these drugs during renal ischemia and hemorrhagic shock is contraindicated because by blocking the production of prostaglandins, they decrease RBF and increase renal ischemia. Prostaglandins play an increasingly important role in maintaining RBF and GFR as individuals age. Accordingly, NSAIDs can significantly reduce RBF and GFR in the elderly.
|
NO, an endothelium-derived relaxing factor, is an important vasodilator under basal conditions, and it counteracts the vasoconstriction produced by angiotensin II and catecholamines. When blood flow increases, greater shear force acts on endothelial cells in the arterioles and increases the production of NO. In addition, a number of vasoactive hormones, including acetylcholine, histamine, bradykinin, and ATP, facilitate the release of NO from endothelial cells. Increased production of NO causes dilation of the afferent and efferent arterioles in the kidneys. Whereas increased levels of NO decrease total peripheral resistance, inhibition of NO production increases total peripheral resistance.
|
page 574 |  | page 575 |
Figure 32-22 Pathway by which hemorrhage activates renal sympathetic nerve activity and stimulates the production of angiotensin II. (Modified from Vander AJ: Renal Physiology, 2nd ed. New York, McGraw-Hill, 1980.) |
Abnormal production of NO is observed in individuals with diabetes mellitus and hypertension. The excess renal NO production in diabetes may be responsible for glomerular hyperfiltration (i.e., increased GFR) and damage to the glomerulus, problems characteristic of this disease. Elevated NO levels increase glomerular capillary pressure secondary to a fall in resistance of the afferent arteriole. The ensuing hyperfiltration is thought to cause glomerular damage. The normal response to an increase in dietary salt intake includes stimulation of renal NO production, which prevents an increase in blood pressure. In some individuals, however, NO production may not increase appropriately in response to an elevation in salt intake, so blood pressure rises. |
 |
Endothelin is a potent vasoconstrictor secreted by endothelial cells of the renal vessels, mesangial cells, and distal tubular cells in response to angiotensin II, bradykinin, epinephrine, and endothelial shear stress. Endothelin causes profound vasoconstriction of the afferent and efferent arterioles and decreases GFR and RBF. Although this potent vasoconstrictor may not influence GFR and RBF in resting subjects, production of endothelin is elevated in a number of glomerular disease states (e.g., renal disease associated with diabetes mellitus).
|
Kallikrein is a proteolytic enzyme produced in the kidneys. Kallikrein cleaves circulating kininogen to bradykinin, which is a vasodilator that acts by stimulating the release of NO and prostaglandins. Bradykinin increases GFR and RBF.
|
Adenosine is produced within the kidneys and causes vasoconstriction of the afferent arteriole, thereby reducing GFR and RBF. As previously mentioned, adenosine may play a role in tubuloglomerular feedback.
|
Secretion of atrial natriuretic peptide (ANP) by the cardiac atria and brain natriuretic peptide (BNP) by the cardiac ventricle increases when extracellular fluid volume is expanded. Both ANP and BNP dilate the afferent arteriole and constrict the efferent arteriole. Therefore, ANP and BNP produce a modest increase in GFR with little change in RBF.
|
page 575 |  | page 576 |
Figure 32-23 Examples of the interactions of endothelial cells with smooth muscle and mesangial cells. ACE, angiotensin-converting enzyme; AI, angiotensin I; AII, angiotensin II. (Modified from Navar LG et al: Physiol Rev 76:425, 1996.) |
Cells release ATP into the renal interstitial fluid. ATP has dual effects on GFR and RBF. Under some conditions, ATP constricts the afferent arteriole, reduces RBF and GFR, and may play a role in tubuloglomerular feedback. In contrast, ATP may stimulate NO production and increase GFR and RBF.
|
Administration of therapeutic doses of glucocorticoids increases GFR and RBF.
|
The local release of histamine modulates RBF during the resting state and during inflammation and injury. Histamine decreases the resistance of the afferent and efferent arterioles and thereby increases RBF without elevating GFR.
|
The proximal tubule produces the vasodilator substance dopamine. Dopamine has several actions within the kidney, such as increasing RBF and inhibiting renin secretion.
|
Finally, as illustrated in Figure 32-23, endothelial cells play an important role in regulating the resistance of the renal afferent and efferent arterioles by producing a number of paracrine hormones, including NO, prostacyclin (PGI2), endothelin, and angiotensin II. These hormones regulate contraction or relaxation of smooth muscle cells in afferent and efferent arterioles and mesangial cells. Shear stress, acetylcholine, histamine, bradykinin, and ATP stimulate the production of NO, which increases GFR and RBF. Angiotensin-converting enzyme (ACE), located on the surface of endothelial cells lining the afferent arteriole and glomerular capillaries, converts angiotensin I to angiotensin II, which decreases GFR and RBF. Angiotensin II is also produced locally in granular cells in the afferent arteriole and proximal tubular cells. Secretion of PGI2 and PGE2 by endothelial cells, stimulated by sympathetic nerve activity and angiotensin II, increases GFR and RBF. Finally, the release of endothelin from endothelial cells decreases GFR and RBF.
|
ACE degrades and thereby inactivates bradykinin, and it converts angiotensin I, an inactive hormone, to angiotensin II, an active hormone. Thus, ACE increases angiotensin II levels and decreases bradykinin levels. Drugs called ACE inhibitors (e.g., enalapril, captopril), which reduce systemic blood pressure in patients with hypertension, decrease angiotensin II levels and elevate bradykinin levels. Both effects lower systemic vascular resistance, reduce blood pressure, and decrease renal vascular resistance, thereby increasing GFR and RBF. Angiotensin II receptor antagonists (e.g., losartan) are also used to treat high blood pressure. As their name suggests, they block the binding of angiotensin II to the angiotensin II receptor (AT1). These antagonists block the vasoconstrictor effects of angiotensin II on the afferent arteriole; thus, they increase GFR and RBF. In contrast to ACE inhibitors, angiotensin II receptor antagonists do not inhibit kinin metabolism (e.g., bradykinin). |
 |
page 576 |  | page 577 |
- The first step in urine formation is the passive movement of a plasma ultrafiltrate from the glomerular capillaries into Bowman's space. The term ultrafiltration refers to the passive movement of an essentially protein-free fluid from the glomerular capillaries into Bowman's space. The endothelial cells of glomerular capillaries are covered by a basement membrane that is surrounded by podocytes. The capillary endothelium, basement membrane, and foot processes of podocytes form the so-called filtration barrier.
- The juxtaglomerular apparatus is one component of an important feedback mechanism (i.e., tubuloglomerular feedback) that regulates RBF and GFR. The structures that make up the juxtaglomerular apparatus include the macula densa, extraglomerular
mesangial cells, and renin- and angiotensin II-producing granular cells.
- Clinically, the GFR is evaluated by measuring plasma [creatinine].
- Autoregulation allows GFR and RBF to remain constant despite changes in arterial blood pressure between 90 and 180 mm Hg. Sympathetic nerves, catecholamines, angiotensin II, prostaglandins, NO, endothelin, natriuretic peptides, bradykinin, and adenosine exert substantial control over GFR and RBF.
|
 |
|